Negatively Charged Particles: Uses & Examples

Negatively charged particles, fundamental constituents of matter, exhibit properties crucial to understanding the microscopic world and macroscopic phenomena. Electrons, as a common example of a negatively charged particle, mediate chemical bonding and electrical conductivity in materials. Cathode rays, streams of high-speed electrons discovered in the late 19th century, exhibit properties that enabled J.J. Thomson to determine the charge-to-mass ratio of the negatively charged particle, i.e., the electron. Applications of negatively charged particles can be found in the semiconductor industry, in particular, doping semiconductors with elements such as phosphorus introduces additional electrons, thereby modifying their electrical characteristics.
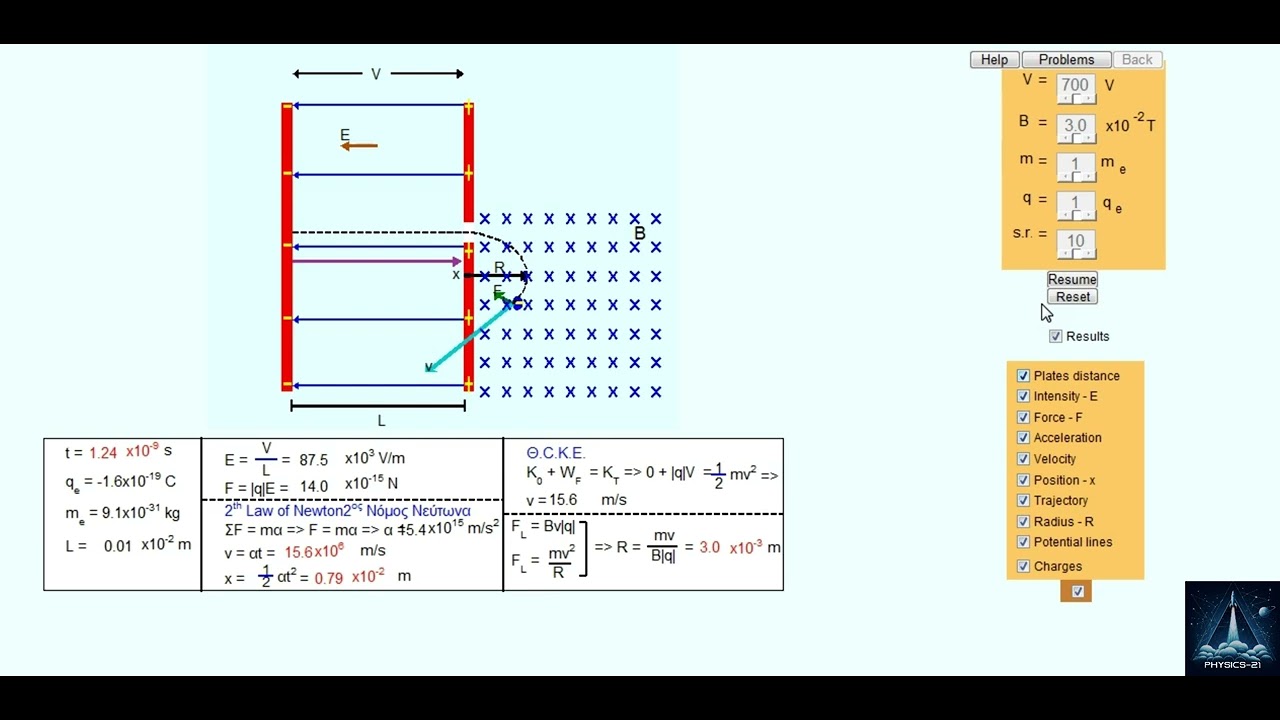
Image taken from the YouTube channel Physics-21 , from the video titled Electromagnetism Guide: Sequential Motion of a Negatively Charged Particle Tutorial .
The Unseen Power of Negative Charge Carriers
Negative charge, an intrinsic property of matter, is far more than a mere scientific abstraction.
It is the invisible architect of our reality, the fundamental force shaping everything from the smallest atoms to the most complex technological marvels.
This exploration delves into the ubiquitous realm of negative charge carriers, elucidating their properties, diverse forms, and the profound impact they wield across various scientific disciplines.
Understanding the Fundamentals of Negative Charge
At its core, negative charge is one of two types of electrical charge, the other being positive.
These charges interact through the electromagnetic force: like charges repel, while opposite charges attract.
This fundamental interaction governs the structure of atoms, the flow of electricity, and the very fabric of chemical bonding.
Without it, the universe as we know it could not exist.
The magnitude of electric charge is quantized, meaning it exists in discrete units.
The smallest unit of free charge is the elementary charge, 'e', which is carried by elementary particles like the electron.
Scope of the Exploration: A Journey Through Charge Carriers
This article will focus on the primary carriers of negative charge, examining their individual properties and collective influence.

We will investigate fundamental particles, such as electrons and quarks, along with their roles in atomic structure and particle physics.
The behaviour of negative charge carriers also influences the state of matter. For example, in plasmas where electrons are freely moving.
Beyond fundamental physics, we will explore the technological applications that rely on precise control of negative charge.
These applications range from batteries and semiconductors to advanced imaging techniques.
A Historical Glimpse: Unraveling the Mystery of Charge
The understanding of negative charge has evolved over centuries of scientific inquiry.
Early experiments with static electricity revealed the existence of two distinct types of charge, later labeled positive and negative by Benjamin Franklin.
However, it was not until the late 19th and early 20th centuries that the true nature of negative charge began to emerge, with groundbreaking experiments led by scientists such as J.J. Thomson, who is credited with discovering the electron.
Robert Millikan's oil drop experiment then precisely quantified the elementary charge, solidifying the electron's role as a fundamental carrier of negative charge.
These historical milestones paved the way for our modern understanding of electromagnetism and quantum mechanics, revealing the central role of negative charge in the universe.
The Fundamental Particles: Leptons and Quarks—Nature's Tiny Carriers
The Unseen Power of Negative Charge Carriers Negative charge, an intrinsic property of matter, is far more than a mere scientific abstraction. It is the invisible architect of our reality, the fundamental force shaping everything from the smallest atoms to the most complex technological marvels. This exploration delves into the ubiquitous realm of negative charge carriers, beginning with the most fundamental particles that bear this essential property.
These elementary particles, leptons and quarks, are the indivisible building blocks of matter, dictating the interactions and characteristics of the universe at its smallest scales. Understanding these particles is crucial to grasping the nature of negative charge and its profound implications.
Leptons: The Lightweight Champions of Negative Charge
Leptons are a family of fundamental particles that do not experience the strong nuclear force. Among these, three carry a negative charge: the electron, the muon, and the tau.
The Electron: Ubiquitous and Essential
The electron (e⁻) stands as the most well-known and practically relevant negative charge carrier. Its discovery by J.J. Thomson in 1897 revolutionized our understanding of the atom, revealing it as a composite structure rather than an indivisible entity.
With a negative charge of -1e (where e is the elementary charge), the electron is the primary charge carrier in most everyday electrical phenomena. Its remarkably small mass and stability render it indispensable in chemical bonding, electrical conductivity, and countless technological applications.
Muons and Taus: Unstable Heavyweights
The muon (μ⁻) and tau (τ⁻) are heavier, unstable cousins of the electron. They possess the same negative charge as the electron but significantly greater masses, leading to their classification as second- and third-generation leptons, respectively.
These particles are primarily observed in high-energy physics experiments, such as those conducted at particle accelerators like the Large Hadron Collider (LHC). Due to their instability, muons and taus rapidly decay into other particles, providing valuable insights into the fundamental forces and interactions governing the universe.
Their relatively short lifespans—on the order of microseconds for muons and even shorter for taus—limit their direct technological applications compared to the electron. However, their decay processes and interactions with other particles are essential for testing the Standard Model of particle physics.
Quarks: Confined Carriers of Fractional Charge
Quarks are fundamental constituents of matter that do experience the strong nuclear force, in addition to the electromagnetic, weak, and gravitational forces. Unlike leptons, quarks are never observed in isolation.
They are always bound together to form composite particles called hadrons, such as protons and neutrons. Of the six known types of quarks, three carry a negative charge: down, strange, and bottom.
Fractional Charge and Confinement
A unique characteristic of these quarks is their fractional electric charge of -1/3 e. This contrasts sharply with the integer charge of leptons.
The strong force binds these quarks within hadrons so tightly that isolating a single quark requires an infinite amount of energy. This phenomenon, known as color confinement, ensures that quarks are always found in combinations that result in a net color charge of zero, analogous to electrical neutrality.
The Role in Hadrons
The charge of quarks determines the overall charge of the hadrons they comprise. For instance, a proton, with a charge of +1e, is composed of two up quarks (+2/3 e each) and one down quark (-1/3 e), resulting in a net charge of +1e.
Similarly, a neutron, with a charge of 0, consists of one up quark (+2/3 e) and two down quarks (-1/3 e each), leading to a net charge of 0. Thus, the fractional negative charge of down, strange, and bottom quarks plays a crucial role in establishing the properties of protons and neutrons, the very building blocks of atomic nuclei.
Ions: Gaining Electrons, Becoming Anions
Beyond fundamental particles, negatively charged ions, or anions, are essential charge carriers in chemistry and biology. Anions form when an atom or molecule gains one or more electrons, resulting in a net negative charge.
Formation and Significance
The formation of anions is driven by the tendency of atoms to achieve a stable electron configuration, often resembling that of a noble gas. Elements like chlorine and oxygen readily gain electrons to form chloride (Cl⁻) and oxide (O²⁻) ions, respectively.
These ions play crucial roles in a wide range of chemical reactions, including acid-base reactions, redox reactions, and the formation of ionic compounds like sodium chloride (NaCl). In biological systems, anions such as chloride, bicarbonate (HCO₃⁻), and phosphate (PO₄³⁻) are vital for maintaining pH balance, regulating nerve impulses, and facilitating enzyme activity.
Fundamental Concepts: The Laws Governing Negative Charge
Understanding the behavior of negative charge carriers necessitates an exploration of the core concepts that govern their interactions. These concepts, including electricity, electric charge, electromagnetism, and electric fields, are the bedrock upon which our understanding of the physical world is built. They dictate the forces at play and the interactions that shape the behavior of negatively charged particles.
Electricity and Electric Charge: The Foundation
Electricity, at its most fundamental, is the physical property that causes matter to experience a force when placed in an electromagnetic field. It is an intrinsic characteristic of certain subatomic particles, most notably the electron.
Electric charge, the quantitative measure of electricity, exists in discrete units. This discreteness leads to the concept of quantization of electric charge, meaning that any observable charge is an integer multiple of the elementary charge.
The concept of the elementary charge is fundamental to understanding the nature of electric charge. It represents the smallest unit of charge that can exist freely and is denoted by the symbol 'e'.
Elementary Charge (e): The Unit of Measurement
The elementary charge (e) is a fundamental physical constant, representing the magnitude of charge carried by a single proton or electron. Its precise value is approximately 1.602 × 10⁻¹⁹ Coulombs.
This value serves as the benchmark for measuring the strength of electromagnetic interactions. It is not merely a numerical constant; it reflects the inherent structure of the universe at the quantum level.
The elementary charge is crucial in determining the magnitude of forces and energies involved in electromagnetic interactions, playing a key role in calculations related to atomic and molecular behavior.
Electromagnetism: The Force in Action
Electromagnetism is the fundamental force governing the interactions between charged particles. It encompasses both electric and magnetic phenomena, unified into a single, coherent force field.
This force is mediated by photons, massless particles that act as the carriers of electromagnetic interaction. The exchange of photons between charged particles results in the attraction or repulsion that we observe.
Electromagnetism is the force responsible for holding atoms and molecules together. Without it, matter as we know it could not exist.
The intricate dance of electrons and photons creates the bonds that form all chemical substances, underlying the vast complexity of the material world.
Electric Field: The Area of Influence
An electric field is the region surrounding a charged particle where other charged particles experience a force. It is a vector field, meaning that it has both magnitude and direction at every point in space.
The strength of the electric field is proportional to the amount of charge producing the field and inversely proportional to the square of the distance from the charge, following Coulomb's Law.
Electric field lines are a useful visual tool for representing electric fields. These lines indicate the direction of the force that a positive test charge would experience if placed in the field.
The density of the field lines is proportional to the strength of the electric field, providing a qualitative measure of the force's intensity. Electric fields are integral to understanding how charged particles interact.
Implications for Matter: How Negative Charge Shapes Our World
Understanding the behavior of negative charge carriers necessitates an exploration of the core concepts that govern their interactions. These concepts, including electricity, electric charge, electromagnetism, and electric fields, are the bedrock upon which our understanding of the physical world is built. But the story doesn't end with fundamental laws; the real intrigue lies in how these principles manifest in the very structure and properties of matter. From the architecture of atoms to the exotic state of plasma, negative charge is a master sculptor.
Atomic Structure: The Electron's Orchestration
At the heart of every atom, negative charge, embodied by electrons, dictates its identity and its interactions with other atoms. These subatomic particles are not simply orbiting a nucleus; they exist in quantized energy levels and orbitals, forming a complex and dynamic system.
Electrons arrange themselves around the nucleus according to the principles of quantum mechanics. Their distribution is not random but rather structured according to specific energy levels and orbitals.
This configuration is critical because it determines an element's chemical properties. For example, atoms with nearly full or nearly empty outer electron shells are highly reactive, seeking to achieve stability by gaining or losing electrons.
This drive to achieve a stable electron configuration is the driving force behind chemical bonding. The dance of electrons dictates the formation of molecules and, consequently, the diverse array of substances that make up our world.
Chemical Properties: The Electron's Fingerprint
The chemical behavior of an element is intrinsically linked to the arrangement of its electrons. Elements in the same group of the periodic table, for instance, share similar chemical properties because they have the same number of valence electrons – those in the outermost shell.
These valence electrons are the key players in chemical reactions, determining how an atom will interact with its neighbors.
The electronegativity of an atom, its ability to attract electrons in a chemical bond, is another crucial property that stems directly from its electron configuration.
Highly electronegative atoms, like oxygen and fluorine, tend to pull electrons towards themselves in a bond, creating polar molecules with uneven charge distributions. This polarity is fundamental to many chemical and biological processes.
Plasma: A Sea of Free Electrons
Moving beyond individual atoms, we encounter plasma, often called the fourth state of matter. Plasma is essentially an ionized gas consisting of free electrons and positively charged ions. This unique state of matter arises at high temperatures, where atoms lose their electrons, creating a soup of charged particles.
The abundance of free electrons gives plasma extraordinary properties. One of the most notable is its high electrical conductivity. Because electrons are free to move, plasma can conduct electricity much more efficiently than a neutral gas.
Plasma is also highly responsive to magnetic fields, with charged particles spiraling around magnetic field lines. This interaction is the basis for many plasma applications, from fusion energy research to industrial processing.
Plasma is not some exotic substance confined to laboratories; it's actually quite common in the universe. Stars, including our Sun, are largely composed of plasma. Lightning is a dramatic example of plasma formation in our atmosphere. The Northern Lights, or Aurora Borealis, is caused by charged particles from the Sun interacting with the Earth's magnetic field and creating plasma in the upper atmosphere.
In conclusion, negative charge carriers, particularly electrons, are not just abstract particles; they are the architects of matter. Their behavior dictates the structure and properties of atoms, the formation of molecules, and even the existence of exotic states of matter like plasma. Understanding the role of negative charge is, therefore, essential to understanding the world around us.
Technological Applications: Harnessing the Power of Electrons
Understanding the behavior of negative charge carriers allows for manipulation and control, leading to a plethora of technologies that shape modern life. These applications, ranging from energy storage to medical treatments, demonstrate the profound impact of harnessing the power of electrons.
Batteries: Storing Electrical Energy Through Controlled Electron Flow
Batteries are fundamental to modern life, providing portable energy for countless devices. Their operation relies on precisely controlled electrochemical reactions that drive the flow of electrons.
At the heart of a battery are two electrodes (an anode and a cathode) immersed in an electrolyte.
The electrochemical reactions within the battery generate a potential difference, which compels electrons to flow through an external circuit, powering the connected device.
Different types of batteries, such as lithium-ion, nickel-metal hydride, and lead-acid, employ varying chemical reactions and materials, resulting in different performance characteristics, lifespan, and environmental impacts.
Semiconductor Devices: The Foundation of Modern Electronics
Semiconductor devices, like transistors and diodes, are the cornerstone of modern electronics. Their functionality hinges on the precise control of electron flow within semiconductor materials like silicon and germanium.
Transistors act as electronic switches or amplifiers, controlling the flow of electrons to perform logical operations and amplify electronic signals.
Diodes, on the other hand, allow current to flow in only one direction, acting as rectifiers in power supplies and other electronic circuits.
Electron Microscopes: Visualizing the Nanoscale
Electron microscopes, including Transmission Electron Microscopes (TEM) and Scanning Electron Microscopes (SEM), enable scientists to visualize structures at the nanoscale, far beyond the reach of conventional light microscopes.
TEM uses a beam of electrons that passes through a thin sample, creating a high-resolution image based on the electron transmission patterns.
SEM, in contrast, scans a focused electron beam across the surface of a sample, generating an image based on the emitted secondary electrons.
These microscopes are invaluable in materials science, biology, and nanotechnology, allowing researchers to investigate the structure and properties of materials and biological specimens at the atomic level.
X-ray Machines: Illuminating the Invisible
X-ray machines utilize high-energy electrons to generate X-rays, a form of electromagnetic radiation capable of penetrating soft tissues.
X-rays are produced by accelerating electrons to high speeds and bombarding them against a metal target.
The resulting X-rays are then used in medical imaging to visualize bones, organs, and other internal structures, aiding in the diagnosis of various medical conditions.
In industrial settings, X-rays are employed for non-destructive testing, allowing engineers to inspect welds, detect flaws in materials, and ensure the quality of manufactured products.
Solar Cells: Converting Sunlight into Electricity
Solar cells, also known as photovoltaic cells, harness the energy of sunlight to generate electricity. This process relies on the photoelectric effect, where photons of light excite electrons in a semiconductor material, creating an electric current.
Common materials used in solar cells include silicon, cadmium telluride, and perovskites.
The efficiency of solar cells in converting sunlight into electricity varies depending on the material and design, with ongoing research focused on improving efficiency and reducing costs to make solar energy more accessible.
Electroplating: Coating with Precision
Electroplating is a process that uses electricity to deposit a thin layer of metal onto a conductive surface.
The object to be plated is immersed in an electrolyte solution containing ions of the metal to be deposited, and an electric current is passed through the solution.
The metal ions are reduced at the surface of the object, forming a thin, adherent coating.
Electroplating is widely used in manufacturing for corrosion protection, wear resistance, and decorative purposes, enhancing the properties and appearance of various products.
Medical Imaging: X-rays and CT Scans - A Window into the Human Body
X-ray machines, previously mentioned, are crucial components of medical imaging. The ability of X-rays to penetrate tissue allows doctors to visualize bones and detect abnormalities within the body.
Computed Tomography (CT) scans utilize X-rays to create detailed cross-sectional images of the body, providing a three-dimensional view of internal organs, bones, and blood vessels.
These imaging techniques are invaluable for diagnosing a wide range of medical conditions, from fractures to tumors.
Radiation Therapy: Targeting Cancer with Electrons
Radiation therapy uses high-energy electrons or X-rays to destroy cancer cells.
The focused radiation damages the DNA of cancer cells, preventing them from growing and dividing.
Radiation therapy can be delivered externally, using a machine that directs radiation beams at the tumor, or internally, using radioactive implants placed near the tumor. The precision of modern radiation therapy techniques minimizes damage to surrounding healthy tissues, improving patient outcomes.
Pioneers of Discovery: Scientists Who Unveiled the Secrets of Negative Charge
Understanding the behavior of negative charge carriers allows for manipulation and control, leading to a plethora of technologies that shape modern life. Before these applications could become reality, however, pioneering scientists had to unravel the fundamental nature of negative charge itself. This section acknowledges the monumental contributions of J.J. Thomson and Robert Millikan, whose work laid the groundwork for our modern understanding of the electron.
J. Thomson: Unveiling the Electron's Existence
J.J. Thomson's discovery of the electron in 1897 was a watershed moment in physics.
His experiments with cathode ray tubes demonstrated that these rays were composed of negatively charged particles, a previously unknown constituent of the atom.
The Cathode Ray Tube Experiments
Thomson's meticulous experiments involved manipulating cathode rays using electric and magnetic fields.
By carefully measuring the deflection of the rays, he was able to determine the charge-to-mass ratio of the particles.
This ratio was constant, regardless of the gas used in the tube, suggesting that these particles were a fundamental component of all atoms.
Significance of Thomson's Discovery
Thomson's work revolutionized our understanding of atomic structure.
Prior to his discovery, atoms were thought to be indivisible.
His findings proved that atoms were, in fact, composite structures containing negatively charged subatomic particles.
This discovery not only earned Thomson the Nobel Prize in Physics in 1906, but also fundamentally altered the course of physics and chemistry.
Early Atomic Models: The Plum Pudding Model
Based on his findings, Thomson proposed the "plum pudding" model of the atom.
This model envisioned the atom as a sphere of positive charge with negatively charged electrons embedded within it, much like plums in a pudding.
While ultimately incorrect, the plum pudding model was a crucial step in the development of more accurate atomic models.
It stimulated further research and laid the foundation for future discoveries about the structure of matter.
Robert Millikan: Quantifying the Electron's Charge
While Thomson determined the charge-to-mass ratio of the electron, Robert Millikan's oil drop experiment provided the definitive measurement of the electron's charge.
Millikan's ingenious experiment allowed scientists to precisely quantify this fundamental constant of nature.
The Oil Drop Experiment
In Millikan's experiment, tiny oil droplets were sprayed into a chamber and observed through a microscope.
The droplets were electrically charged by exposing them to ionizing radiation.
By carefully adjusting the electric field in the chamber, Millikan could suspend the charged oil droplets against the force of gravity.
By measuring the electric field required to suspend the droplets and knowing the mass of the droplets, he could calculate the electric charge on each droplet.
The Quantization of Electric Charge
Millikan's most significant finding was that the charge on each oil droplet was always a multiple of a fundamental unit of charge.
This demonstrated that electric charge is quantized, meaning it exists only in discrete units.
This smallest unit of charge was identified as the charge of a single electron.
His precise determination of this value, approximately 1.602 x 10^-19 coulombs, is a cornerstone of modern physics.
The Legacy of Millikan's Work
Millikan's oil drop experiment provided unambiguous proof of the existence of elementary electric charge and allowed for its precise measurement.
This accomplishment earned him the Nobel Prize in Physics in 1923.
His work has had a lasting impact on our understanding of the fundamental constants of nature.
The accurate value of the electron's charge is essential for numerous calculations in physics, chemistry, and engineering, underpinning countless technological applications.
Video: Negatively Charged Particles: Uses & Examples
Negatively Charged Particles: FAQs
What are some common examples of negatively charged particles?
Electrons are the most well-known negatively charged particle. Other examples include negatively charged ions like chloride (Cl-) which has gained an extra electron. Muons are another type of negatively charged particle, though less common in everyday experience.
How are negatively charged particles used in medical imaging?
In X-ray imaging, electrons, as negatively charged particles, are accelerated and directed at a target. These electrons interact with the target material, producing X-rays that can then be used to create images of bones and tissues.
Why are negatively charged particles important in chemical bonding?
Chemical bonds often involve the sharing or transfer of electrons, which are negatively charged particles. This interaction of negative charges between atoms leads to the formation of molecules and compounds that make up all matter around us.
How do negatively charged particles contribute to electricity?
Electricity fundamentally relies on the flow of negatively charged particles, primarily electrons. The movement of these negatively charged particles through a conductor creates an electric current, powering our devices and lighting our homes.
So, the next time you're zapping static cling or marveling at a lightning storm, remember the tiny, but mighty, negatively charged particle at the heart of it all. From powering our homes to revolutionizing medicine, these little guys are doing some seriously big work, and that's definitely something to think about!