Transverse vs Longitudinal Waves Explained
The fundamental characteristic of waves involves the transfer of energy, but understanding the distinction hinges on wave type. This difference is most evident when one tries to explain between a transverse wave and longitudna wave. Specifically, a transverse wave, like those studied in seismology at the San Andreas Fault, exhibits oscillations perpendicular to its direction of travel, contrasting sharply with longitudinal waves. Conversely, a longitudinal wave, such as a sonic boom from a supersonic aircraft, oscillates parallel to its direction of travel.
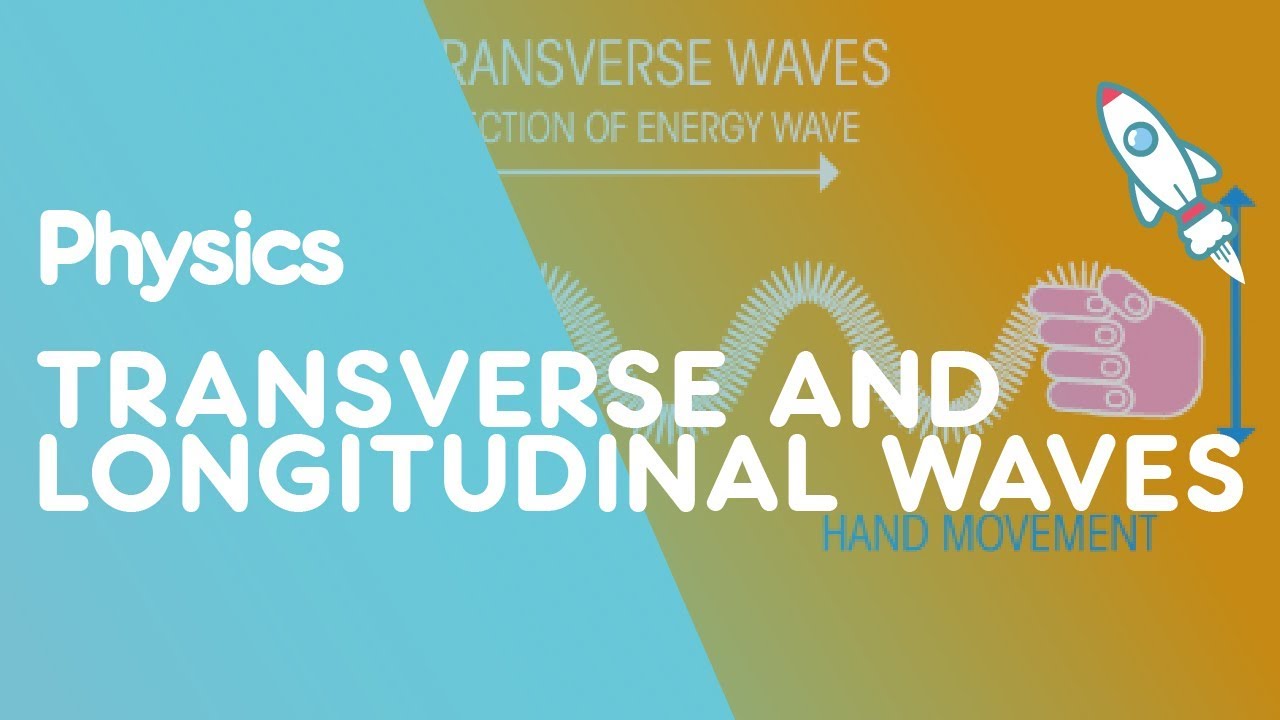
Image taken from the YouTube channel FuseSchool - Global Education , from the video titled Transverse & Longitudinal Waves | Waves | Physics | FuseSchool .
At its core, a wave is a disturbance that propagates through a medium or even space, transferring energy from one location to another. This energy transfer occurs without the wholesale movement of the medium itself. Imagine dropping a pebble into a pond: the ripples that spread outwards are waves, carrying energy across the water's surface, though the water molecules themselves primarily oscillate locally.
The Ubiquity of Wave Principles
The principles governing wave behavior are not confined to a single area of study. They are, in fact, foundational to numerous scientific and technological disciplines. Physics, engineering, and medicine all rely heavily on understanding wave phenomena to develop new technologies and deepen our understanding of the natural world.
From the design of noise-canceling headphones to the development of advanced medical imaging techniques, waves play a pivotal role. Neglecting the fundamental principles of wave mechanics would severely limit innovation and progress in these critical fields.
Waves in Everyday Technology
Consider the technologies we use daily. Communication systems, for instance, rely on electromagnetic waves to transmit information across vast distances. Radio waves, microwaves, and even visible light are all forms of electromagnetic radiation that adhere to wave principles.
Communication and Imaging
Similarly, medical imaging techniques like ultrasound utilize sound waves to create detailed images of the human body. The way these waves interact with different tissues provides valuable diagnostic information.
These examples illustrate just how deeply ingrained wave phenomena are in the technologies that shape our modern lives. Without a thorough understanding of waves, the development and refinement of these technologies would be significantly hampered.
Transverse vs. Longitudinal Waves: A Comparative Analysis
At its core, a wave is a disturbance that propagates through a medium or even space, transferring energy from one location to another.
This energy transfer occurs without the wholesale movement of the medium itself.
Imagine dropping a pebble into a pond: the ripples that spread outwards are waves, carrying energy across the water's surface, though the water itself largely stays put.
While all waves share this fundamental characteristic, they manifest in diverse forms, notably as transverse and longitudinal waves.
Understanding the distinction between these two wave types is crucial to grasping their behavior in various physical phenomena.
Transverse Waves: Oscillation Perpendicular to Propagation
Transverse waves are characterized by the oscillation of particles perpendicular to the direction in which the wave is traveling.
Imagine shaking a rope up and down; the wave travels along the rope, but the rope itself moves vertically.
Examples of Transverse Waves
Light waves are a prime example of transverse waves.
They are electromagnetic in nature and do not require a medium to propagate, allowing them to travel through the vacuum of space.
S-waves, or shear waves, generated by earthquakes, are another example.
These seismic waves can only travel through solids, as fluids cannot support shear stress.
Water waves, while complex, also exhibit transverse characteristics, particularly in the vertical motion of the water surface.
Crests and Troughs: Defining Features
The key characteristics of transverse waves are their crests (the highest points) and troughs (the lowest points).
These represent the maximum positive and negative displacements of the medium, respectively, from its equilibrium position.
The distance between two successive crests or troughs defines the wavelength of the transverse wave.
Longitudinal Waves: Oscillation Parallel to Propagation
In contrast to transverse waves, longitudinal waves involve particle oscillation parallel to the direction of wave propagation.
Picture pushing and pulling a Slinky; the compressions and rarefactions travel along the Slinky in the same direction as your push and pull.
Examples of Longitudinal Waves
Sound waves are a quintessential example of longitudinal waves.
They consist of compressions (regions of high pressure) and rarefactions (regions of low pressure) traveling through a medium like air.
P-waves, or primary waves, generated by earthquakes, are also longitudinal.
These seismic waves can travel through solids, liquids, and gases, making them the first to be detected by seismographs after an earthquake.
Compressional waves in a spring, as described earlier, provide a tangible model of longitudinal wave behavior.
Compressions and Rarefactions: Defining Features
Longitudinal waves are characterized by compressions, where the medium is compressed, and rarefactions, where the medium is expanded.
These regions of high and low density propagate along the direction of the wave.
The distance between two successive compressions or rarefactions defines the wavelength of the longitudinal wave.
Comparative Analysis: Key Differences and Similarities
The most fundamental distinction between transverse and longitudinal waves lies in the direction of particle oscillation relative to the wave's direction of travel.
In transverse waves, the oscillation is perpendicular, while in longitudinal waves, it is parallel.
Medium Requirements: A Crucial Consideration
Another important difference is their ability to propagate through different media.
Transverse waves, like light, can propagate through a vacuum, as they do not rely on particle interaction.
Longitudinal waves, however, typically require a medium to propagate, as they rely on the compression and rarefaction of particles.
Sound, for example, cannot travel through the vacuum of space.
Additional Real-World Examples
Beyond the examples already mentioned, consider radio waves, which are transverse electromagnetic waves used for communication.
In contrast, consider ultrasound, which utilizes longitudinal sound waves for medical imaging.
Understanding the specific characteristics of each wave type allows us to harness their properties for diverse technological applications, from communication and imaging to earthquake detection and material analysis.
Key Properties of Waves: Amplitude, Wavelength, Frequency, Speed, and Period
Understanding the distinction between transverse and longitudinal waves is crucial, but equally important is grasping the quantitative characteristics that define wave behavior. These properties—amplitude, wavelength, frequency, wave speed, and period—provide the essential tools for analyzing and predicting how waves interact with their environment. They form the bedrock of wave mechanics.
Amplitude: The Measure of a Wave's Strength
Amplitude, in its simplest form, is the maximum displacement of a wave from its equilibrium or rest position. It essentially quantifies the intensity of the wave's disturbance.
For a transverse wave, this is visualized as the height of a crest or the depth of a trough relative to the undisturbed medium. For a longitudinal wave, amplitude reflects the degree of compression or rarefaction.
The amplitude is directly related to the energy a wave carries. A higher amplitude corresponds to a greater amount of energy. Think of a loud sound versus a quiet whisper: the loud sound wave has a significantly larger amplitude, delivering more energy to your ear.
Wavelength: The Spatial Extent of a Cycle
Wavelength (λ), is a spatial property, defines the distance over which the wave's shape repeats. It's commonly measured as the distance between two successive crests or troughs in a transverse wave.
Similarly, it is the distance between compressions or rarefactions in a longitudinal wave.
Wavelength is typically measured in meters (m), representing the physical length of one complete wave cycle. The Greek letter lambda (λ) is used to denote it in mathematical formulas.
Frequency: The Rate of Oscillation
Frequency (f) is a temporal property, describes the number of complete wave cycles that pass a given point per unit of time. In essence, it measures how rapidly the wave oscillates.
The standard unit of frequency is Hertz (Hz), where 1 Hz signifies one cycle per second. A wave with a frequency of 10 Hz completes 10 cycles every second.
The symbol 'f' represents frequency in equations. High-frequency waves oscillate rapidly, while low-frequency waves oscillate more slowly.
Wave Speed: How Fast the Disturbance Travels
Wave speed (v) defines the rate at which the wave propagates through a medium. It's a measure of how quickly the energy carried by the wave travels from one location to another.
Wave speed is intrinsically linked to both frequency and wavelength. The relationship is expressed by the fundamental equation: v = fλ.
This equation reveals that wave speed is the product of frequency and wavelength. If you know two of these variables, you can readily calculate the third.
Period: The Duration of a Single Cycle
The period (T) of a wave is the time required for one complete wave cycle to pass a given point. It's the inverse of frequency.
The relationship between period and frequency is expressed as: T = 1/f.
Period is measured in seconds (s). A wave with a high frequency will have a short period, and vice versa.
Interrelationships and Applications
These five properties aren't isolated; they are interconnected and fundamental to understanding wave behavior. For example, altering the frequency of a wave will directly impact its wavelength if the wave speed remains constant.
These concepts are vital in various fields. In telecommunications, understanding frequency and wavelength allows engineers to design efficient communication systems. In medicine, these properties are crucial for interpreting ultrasound images and understanding radiation therapy.
Wave Phenomena in Action: Examples from Sound to Seismic Activity
Understanding the distinction between transverse and longitudinal waves is crucial, but equally important is grasping the quantitative characteristics that define wave behavior. These properties—amplitude, wavelength, frequency, wave speed, and period—provide the essential foundation for analyzing wave behavior in the real world. Now, we turn our attention to how waves manifest in various scenarios, from the sound we hear to the seismic activity that shapes our planet.
Sound Waves: The Mechanics of Hearing
Sound waves offer an excellent example of longitudinal waves in action. Unlike light waves, which can travel through the vacuum of space, sound requires a medium—such as air, water, or solids—to propagate.
The mechanism of sound involves compressions and rarefactions of the medium, creating pressure variations that our ears interpret as sound. The speed of sound is not constant; it depends heavily on the medium through which it travels. Sound waves travel faster through solids than liquids, and faster through liquids than gases.
For example, at room temperature, sound travels at approximately 343 meters per second in air, but in water, that speed increases to around 1481 meters per second.
Light Waves: Electromagnetic Radiation
Light waves stand in stark contrast to sound waves, showcasing the behavior of transverse electromagnetic waves.
Light doesn't need a medium to travel; it can propagate through the vacuum of space. This is how sunlight reaches Earth. A fundamental constant in physics is the speed of light in a vacuum, denoted as c, approximately equal to 3 x 10^8 meters per second. This speed remains constant regardless of the motion of the source or observer, a cornerstone of Einstein's theory of special relativity.
Seismic Waves: Unveiling Earth's Interior
Seismic waves provide invaluable insight into the Earth's internal structure. Generated by earthquakes, volcanic eruptions, and even human-induced explosions, seismic waves come in two primary forms: P-waves (Primary waves) and S-waves (Secondary waves).
P-waves are longitudinal, meaning they can travel through both solid and liquid mediums.
S-waves, on the other hand, are transverse and can only travel through solids. The behavior of these waves as they pass through the Earth helps seismologists map the different layers and compositions of our planet. For instance, the absence of S-waves beyond a certain depth indicates the presence of a liquid outer core.
Water Waves: A Symphony of Motion
Water waves are more complex than simple transverse or longitudinal waves; they exhibit a combination of both behaviors, especially in deep water. Near the surface, water waves appear primarily transverse, with the water particles moving up and down as the wave passes. However, beneath the surface, water particles also exhibit a circular or elliptical motion that has a longitudinal component.
The behavior of water waves is influenced by factors such as water depth, wind speed, and the presence of obstacles. They showcase wave phenomena such as diffraction, refraction, and interference, making them a rich area of study in fluid dynamics.
Understanding these diverse examples—from the sound waves we hear to the seismic waves that shape our understanding of Earth—highlights the pervasive and critical role of waves in our world.
Tools and Instruments for Studying Waves: Visualizing and Demonstrating
Understanding the distinction between transverse and longitudinal waves is crucial, but equally important is grasping the quantitative characteristics that define wave behavior. These properties—amplitude, wavelength, frequency, wave speed, and period—provide the essential foundation for both analyzing and visualizing waves, turning abstract concepts into tangible representations. Let's look at some common tools.
This section delves into specific instruments and their utility in bringing wave phenomena to life.
Oscilloscopes: Capturing Waveforms in Real-Time
The oscilloscope stands as a cornerstone in the field of wave analysis, a powerful tool for visualizing electrical signals that vary with time.
At its core, an oscilloscope plots voltage as a function of time, providing a graphical representation of the waveform.
This allows engineers and scientists to directly observe the amplitude, frequency, and shape of a wave, revealing critical information about the signal's characteristics.
Applications of Oscilloscopes
Oscilloscopes find extensive applications in diverse fields.
In electronics, they are essential for debugging circuits, verifying signal integrity, and measuring signal parameters.
They are also utilized in scientific research to study various wave phenomena, from sound waves to electromagnetic radiation.
Furthermore, oscilloscopes are indispensable in educational settings, providing students with hands-on experience in visualizing and analyzing waveforms.
Digital vs. Analog Oscilloscopes: A Brief Comparison
While both digital and analog oscilloscopes serve the same fundamental purpose, they differ in their method of operation and capabilities.
Analog oscilloscopes use electron beams to directly display waveforms on a screen, offering a real-time view of the signal.
Digital oscilloscopes, on the other hand, sample the input signal and convert it into digital data, which is then processed and displayed on a screen.
Digital oscilloscopes offer advantages such as greater accuracy, storage capabilities, and advanced analysis features.
They’re also more versatile for capturing transient or complex waveforms.
Slinkies and Springs: Hands-On Wave Demonstrations
While sophisticated instruments like oscilloscopes provide precise measurements and visualizations, simpler tools can also be invaluable for understanding wave behavior.
The humble slinky or spring serves as an excellent tool for physically demonstrating transverse and longitudinal waves.
Demonstrating Transverse Waves
By holding one end of the slinky and shaking the other end side-to-side, you can create a transverse wave.
Observe how the disturbance propagates along the slinky perpendicular to the direction of motion.
This vividly illustrates the key characteristic of transverse waves: the oscillation is perpendicular to the direction of wave propagation.
Demonstrating Longitudinal Waves
To demonstrate longitudinal waves, hold one end of the slinky and push and pull the other end in the same direction as the slinky's length.
Observe how compressions and rarefactions propagate along the slinky.
This illustrates the key characteristic of longitudinal waves: the oscillation is parallel to the direction of wave propagation.
Advantages of Slinky Demonstrations
Slinky demonstrations offer several advantages.
They provide a hands-on, intuitive way to understand wave behavior, making abstract concepts more concrete.
They are also relatively inexpensive and accessible, making them ideal for educational purposes.
Moreover, they allow for direct observation of wave properties such as amplitude, wavelength, and speed, enhancing comprehension and engagement.
By combining sophisticated instruments like oscilloscopes with simple tools like slinkies, we can gain a comprehensive understanding of wave phenomena.
Fields of Study Dedicated to Waves: Exploring Related Disciplines
Understanding the distinction between transverse and longitudinal waves is crucial, but equally important is grasping the quantitative characteristics that define wave behavior. These properties—amplitude, wavelength, frequency, wave speed, and period—provide the essential foundation for several fields of study dedicated to unraveling the mysteries of waves.
These fields offer specialized perspectives and applications, building upon core wave principles to address specific phenomena and challenges. Let's explore some of these key disciplines.
Physics: The Foundation of Wave Understanding
Physics serves as the bedrock for understanding waves and their behavior. It delves into the fundamental principles governing wave motion, including wave generation, propagation, and interaction with matter.
Physics provides the theoretical frameworks and mathematical models necessary to describe and predict wave phenomena accurately. It examines not only mechanical waves (like sound and water waves) but also electromagnetic waves (like light and radio waves), offering a comprehensive understanding of wave behavior across the spectrum.
Acoustics: The Science of Sound
Acoustics is the scientific study of sound, encompassing its production, transmission, reception, and effects. It focuses on sound waves, a type of longitudinal wave that propagates through various mediums, including air, water, and solids.
Acoustics explores the properties of sound waves, such as their speed, intensity, and frequency, and how these properties affect human hearing and perception.
This field has diverse applications, ranging from designing concert halls with optimal acoustics to developing noise-canceling technologies and medical ultrasound imaging.
Optics: Illuminating the World with Light
Optics is the branch of physics that studies light and its behavior. It explores the nature of light as an electromagnetic wave, examining its properties, such as reflection, refraction, diffraction, and interference.
Optics plays a vital role in various technologies, including lenses, telescopes, microscopes, lasers, and fiber optics. It also helps us understand how light interacts with matter, leading to advancements in areas like imaging, spectroscopy, and materials science.
Seismology: Unveiling Earth's Secrets through Seismic Waves
Seismology is the scientific study of earthquakes and the propagation of elastic waves through the Earth or other planet-like bodies. It focuses on seismic waves, which are vibrations that travel through the Earth's layers after an earthquake, volcanic eruption, or other significant disturbances.
Seismologists analyze seismic waves to determine the location, magnitude, and mechanism of earthquakes. By studying how seismic waves travel through the Earth, they can also infer the structure and composition of the Earth's interior.
This field is crucial for understanding Earth's dynamic processes and for mitigating the risks associated with earthquakes and tsunamis.
Video: Transverse vs Longitudinal Waves Explained
FAQs: Transverse vs Longitudinal Waves Explained
What's the main difference between how particles move in transverse and longitudinal waves?
In a transverse wave, particles move perpendicular to the wave's direction of travel, like ripples on a pond. In a longitudinal wave, particles move parallel to the wave's direction, bunching up and spreading out, similar to a slinky being pushed and pulled. To explain between a transverse wave and longitudnal, the crucial distinction is the particle motion relative to wave direction.
Can sound travel as a transverse wave?
No, sound cannot travel as a transverse wave in air or fluids. Sound requires a medium where particles can be compressed and expanded. This compression and expansion are parallel to the direction the sound wave travels, making it a longitudinal wave. It is impossible to explain between a transverse wave and longitudnal sound wave because sound waves are longitudinal.
What are some real-world examples of each type of wave?
Light waves are transverse waves, as are radio waves and ripples on water. Sound waves are longitudinal. Other examples include seismic P-waves (longitudinal) and S-waves (transverse) generated during earthquakes. This contrast help to explain between a transverse wave and longitudnal.
Do both wave types require a medium to travel?
Longitudinal waves generally require a medium (like air, water, or a solid) because they rely on the compression and rarefaction of particles. Transverse waves can travel through a medium, or, in the case of electromagnetic waves like light, they can travel through a vacuum. Therefore, it’s important to explain between a transverse wave and longitudnal when considering mediums for travel.
So, the next time you're at a concert and feeling the bass (a longitudinal wave, by the way!) or watching a wave ripple across a pond (that's transverse!), you'll have a better idea of what's going on behind the scenes. Hopefully, this helps clear up the differences between a transverse wave and longitudinal wave, and you can impress your friends with your newfound wave knowledge!