Sodium Bohr Model: Unlocking Atomic Secrets!
The Bohr model, a foundational concept in quantum mechanics, provides a simplified yet powerful framework for understanding atomic structure. Niels Bohr's contribution to atomic theory, specifically the sodium bohr model, elucidates the quantized energy levels of electrons within the sodium atom. Spectral analysis, a critical experimental technique often performed using instruments from companies like Thermo Fisher Scientific, validates the predicted emission lines associated with electron transitions in the sodium bohr model. Therefore, understanding the sodium bohr model provides fundamental insight into electron configuration.
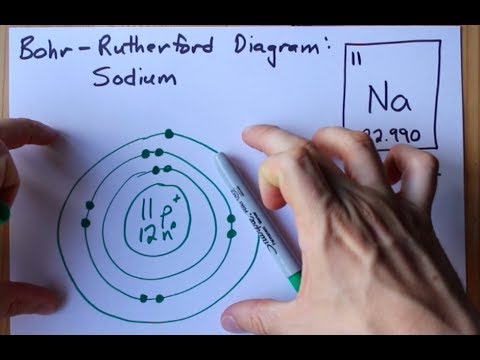
Image taken from the YouTube channel chemistNATE , from the video titled How to Draw the Bohr-Rutherford Diagram of Sodium .
Sodium (Na), an alkali metal with the atomic number 11, is far more than just table salt.
Its implications resonate throughout chemistry, biology, and even industrial processes.
From nerve impulse transmission in our bodies to its role in the production of countless chemicals, Sodium is a cornerstone of both natural and synthetic systems.
Understanding its atomic structure is paramount to comprehending its diverse behavior.
The quest to unravel the atom's mysteries has been a long and winding road, marked by groundbreaking discoveries and paradigm shifts.
A Historical Journey Through Atomic Models
Our understanding of atomic structure has evolved significantly over time.
From Ancient Philosophies to Dalton's Atom
The concept of atoms dates back to ancient Greek philosophers like Democritus, who posited that matter was composed of indivisible units.
However, it was John Dalton's atomic theory in the early 19th century that laid the foundation for modern chemistry.
Dalton proposed that all elements are composed of atoms, which are identical for a given element but differ from those of other elements.
Thomson's Plum Pudding Model
J.J. Thomson's discovery of the electron in 1897 revolutionized atomic theory.
His "plum pudding" model envisioned the atom as a positively charged sphere with negatively charged electrons embedded within it.
Rutherford's Nuclear Model
Ernest Rutherford's gold foil experiment in 1911 led to the development of the nuclear model.
This model proposed that the atom consists of a small, dense, positively charged nucleus surrounded by orbiting electrons.
While a significant advancement, Rutherford's model failed to explain the stability of atoms and the discrete nature of atomic spectra.
The Bohr Model: A Quantum Leap
Niels Bohr's model, proposed in 1913, addressed the shortcomings of Rutherford's model by incorporating quantum concepts.
Bohr postulated that electrons orbit the nucleus in specific energy levels or shells, and that electrons can only transition between these allowed orbits by absorbing or emitting energy in the form of photons.
This model successfully explained the hydrogen atom's spectrum but struggled with more complex atoms.
Decoding Sodium: Purpose and Scope
This article aims to elucidate the Sodium Bohr Model, highlighting its strengths in explaining the element's fundamental properties.
We will explore Sodium's electron configuration, energy levels, and spectroscopic behavior within the framework of the Bohr Model.
We will also underscore the model's significance as a stepping stone in understanding atomic structure and its limitations in light of modern quantum mechanics.
While the Bohr Model provides a simplified picture, it offers valuable insights into the behavior of Sodium and serves as a foundation for more advanced quantum mechanical treatments.
Rutherford's gold foil experiment painted a new picture of the atom, but it also opened up a new set of questions. Namely, what prevents electrons from spiraling into the nucleus, as predicted by classical physics? The answer, or at least a significant step toward it, came from the mind of Niels Bohr.
The Pioneer: Niels Bohr and His Revolutionary Atomic Model
Niels Bohr's atomic model, introduced in 1913, stands as a pivotal moment in the history of quantum mechanics.
It was a daring departure from the established principles of classical physics, proposing revolutionary postulates that reshaped our understanding of the atom.
At its core, the Bohr model introduced the concept of quantized energy levels and defined electron orbits.
Bohr's Revolutionary Postulates
Bohr's model rested on several key postulates, which can be summarized as follows:
-
Electrons occupy specific orbits: Electrons revolve around the nucleus only in certain allowed orbits, without radiating energy.
-
Quantized energy levels: Each orbit corresponds to a specific energy level. Electrons can only possess these discrete energy values.
-
Energy transitions: Electrons can jump from one orbit to another by absorbing or emitting energy in the form of photons. The energy of the photon is equal to the difference in energy between the two orbits.
These postulates defied classical physics, which predicted that electrons orbiting the nucleus should continuously radiate energy.
This would cause them to spiral into the nucleus, resulting in an unstable atom.
A Break from Classical Physics
The Bohr Model was revolutionary because it explicitly broke from classical electromagnetism.
Classical physics could not explain the stability of the atom or the discrete spectral lines observed in atomic emission spectra.
Bohr's postulates, although radical, provided a framework for understanding these phenomena.
By introducing the concept of quantized energy levels, Bohr effectively circumvented the problem of electron collapse.
Electrons could only exist in specific, stable orbits, preventing them from spiraling into the nucleus and radiating energy continuously.
Triumph in Explaining the Hydrogen Spectrum
One of the most significant achievements of the Bohr Model was its ability to accurately predict the line spectrum of hydrogen.
When an electron transitions between energy levels in a hydrogen atom, it emits or absorbs a photon of a specific wavelength.
The Bohr Model successfully predicted these wavelengths, aligning closely with experimental observations.
This predictive power provided strong evidence in support of Bohr's postulates and cemented the model's place in the history of atomic theory.
The Rydberg formula, derived from Bohr's model, accurately calculates the wavelengths of light emitted during transitions between energy levels in hydrogen.
This success was a major triumph for the model.
Despite its limitations (which we will explore later), the Bohr model provided a crucial stepping stone towards the development of more sophisticated quantum mechanical models of the atom.
It laid the groundwork for our modern understanding of atomic structure and behavior.
Rutherford's gold foil experiment painted a new picture of the atom, but it also opened up a new set of questions. Namely, what prevents electrons from spiraling into the nucleus, as predicted by classical physics? The answer, or at least a significant step toward it, came from the mind of Niels Bohr.
Decoding the Sodium Bohr Model: Structure and Configuration
Sodium, a ubiquitous element essential to life and industry, offers a compelling case study for understanding the Bohr model's application to multi-electron atoms. Examining its structure and electron configuration through the lens of Bohr's postulates reveals both the model's explanatory power and its inherent limitations.
Sodium: An Atomic Overview
Sodium (Na) occupies a prominent position in the periodic table, characterized by its atomic number of 11. This number signifies that a neutral sodium atom possesses 11 protons within its nucleus and, consequently, 11 electrons orbiting that nucleus.
The electronic structure of an element describes the arrangement of these electrons, which dictates its chemical behavior. Understanding this arrangement is key to deciphering the properties of sodium.
Unveiling Sodium's Electron Configuration: 2, 8, 1
The Bohr model provides a simplified, yet insightful, framework for understanding electron configuration. According to this model, electrons occupy specific energy levels or shells surrounding the nucleus.
These shells are designated by numbers (n = 1, 2, 3...) or letters (K, L, M...), with the innermost shell (K or n=1) having the lowest energy. Each shell can accommodate a specific maximum number of electrons.
For Sodium, the electron configuration is 2, 8, 1. This means that two electrons occupy the innermost K shell, eight electrons reside in the L shell, and a single, crucial electron occupies the outermost M shell.
Energy Levels and Principal Quantum Numbers
The Bohr model assigns specific energy levels to each electron shell. These energy levels are quantized, meaning electrons can only possess discrete energy values corresponding to the allowed orbits.
The principal quantum number (n) is used to describe these energy levels, with n = 1 representing the K shell, n = 2 representing the L shell, and n = 3 representing the M shell, and so on. Higher values of n correspond to higher energy levels and greater distances from the nucleus.
In the case of sodium, the electron in the M shell (n = 3) possesses a higher energy than the electrons in the L shell (n = 2) or the K shell (n = 1). This energy difference is critical for understanding sodium's spectroscopic properties.
The Valence Electron and Chemical Reactivity
The single electron residing in Sodium's outermost M shell is known as the valence electron. This electron plays a pivotal role in determining sodium's chemical behavior.
Sodium readily loses this valence electron to achieve a stable, filled outer shell configuration. This tendency to lose an electron explains why sodium is highly reactive and readily forms positive ions (Na+).
Sodium and the Alkali Metals
Sodium belongs to the group of elements known as the alkali metals. These elements, located in Group 1 of the periodic table, share similar properties due to their identical valence electron configuration (one electron in their outermost shell).
Alkali metals are characterized by their high reactivity, low ionization energies (the energy required to remove an electron), and tendency to form ionic compounds.
They exhibit properties such as softness, metallic luster when freshly cut, and excellent electrical and thermal conductivity. Sodium, as an alkali metal, exemplifies these characteristic traits.
Decoding the Sodium Bohr Model explained the arrangement of electrons within the atom, dictating its chemical behavior. But how can we experimentally verify these arrangements and energy levels? The answer lies in the unique "fingerprint" each element possesses: its spectrum.
The Spectroscopic Fingerprint: Unveiling Sodium's Unique Spectra
Spectroscopy provides a powerful lens through which we can analyze the composition of matter, including elements like sodium. It allows us to study the interaction of electromagnetic radiation with matter, revealing valuable information about the atom's structure.
At the heart of spectroscopy lies the understanding that atoms absorb and emit light at specific wavelengths. This wavelength specificity creates unique spectral patterns, allowing scientists to identify elements with great accuracy.
Emission Spectrum of Sodium: A Cascade of Light
The emission spectrum is the array of light emitted by an excited atom. When sodium atoms are heated or subjected to electrical discharge, their electrons jump to higher energy levels. These excited electrons are unstable and quickly fall back to lower energy levels.
As an electron transitions to a lower energy level, it releases energy in the form of a photon of light. The energy, and therefore the wavelength, of this photon is precisely defined by the difference in energy between the two levels.
For sodium, the most prominent feature of its emission spectrum is a bright yellow-orange line at approximately 589 nm.
This is actually a doublet, composed of two closely spaced lines, resulting from transitions from two closely spaced excited states to the ground state.
These characteristic spectral lines serve as a unique identifier for sodium, like a fingerprint.
Absorption Spectrum of Sodium: Shadows of Select Wavelengths
Conversely, the absorption spectrum reveals which wavelengths of light are absorbed by an atom. When white light (containing all wavelengths) passes through a sample of sodium vapor, certain wavelengths are selectively absorbed.
These absorbed wavelengths correspond exactly to the wavelengths emitted in the emission spectrum. In other words, sodium atoms absorb light of the same colors that they emit when excited.
The absorption spectrum appears as a continuous spectrum with dark lines at the wavelengths where light has been absorbed by the sodium atoms.
The prominent dark lines in the sodium absorption spectrum coincide with the bright yellow-orange lines in its emission spectrum.
Linking Spectral Lines to Electron Transitions
The beauty of the Bohr model lies in its ability to connect observed spectral lines to specific electron transitions within the atom. Each spectral line corresponds to a specific jump of an electron between two quantized energy levels.
By carefully measuring the wavelengths of the spectral lines, scientists can determine the energy differences between the energy levels in the sodium atom. The energy (E) of the emitted or absorbed photon is related to its wavelength (λ) by the equation:
E = hc/λ
where h is Planck's constant and c is the speed of light.
These energy differences directly correlate to the transitions predicted by the Bohr model's quantized energy levels. This correspondence provided strong evidence for the validity of Bohr's postulates. However, the limitations of the Bohr model prevent it from accurately predicting spectral line intensities or explaining the fine structure observed in more detailed spectra of sodium.
Spectroscopic analysis, while powerful, ultimately reveals the cracks in the foundation of the Sodium Bohr Model. The model, with its neat, well-defined orbits, provides a simplified picture. The reality, as revealed by more advanced experimental techniques, is far more complex. To truly understand the intricacies of sodium and other multi-electron atoms, we must venture beyond Bohr and embrace the more nuanced world of quantum mechanics.
Beyond Bohr: Limitations and the Quantum Mechanical Leap
The Bohr Model, despite its historical significance, suffers from several critical limitations when applied to atoms beyond hydrogen. While it provides a reasonably accurate description of hydrogen's spectrum, its predictions become increasingly inaccurate and even qualitatively incorrect as the number of electrons increases. Sodium, with its eleven electrons, serves as a prime example of where the Bohr Model's shortcomings become glaringly apparent.
The Breakdown of Defined Orbits
One of the most significant limitations of the Bohr Model is its reliance on the concept of fixed, well-defined electron orbits. In reality, electrons do not follow neat, planetary-like paths around the nucleus. Instead, they exist in regions of probability, described by atomic orbitals.
These orbitals represent the likelihood of finding an electron at a particular point in space. This probabilistic nature is a fundamental aspect of quantum mechanics. The Bohr Model cannot account for the complex shapes and spatial distributions of these orbitals.
The Inter-electronic Repulsion Problem
The Bohr Model treats each electron as if it were orbiting the nucleus independently, ignoring the significant repulsive forces between the electrons themselves. In multi-electron atoms like sodium, these electron-electron interactions have a profound impact on the energy levels and behavior of the electrons.
The Bohr Model's inability to incorporate these interactions leads to significant discrepancies between its predictions and experimental observations. The model fails to accurately predict the ionization energy of sodium, as well as the fine structure observed in its spectrum.
Why Quantum Mechanics? Embracing the Wave Nature of Electrons
Quantum mechanics provides a far more accurate and comprehensive description of atomic structure by treating electrons as both particles and waves. This wave-particle duality is a cornerstone of quantum theory. It is described mathematically by the Schrodinger equation.
The Schrodinger equation allows us to calculate the allowed energy levels and the probability distributions (atomic orbitals) of electrons in atoms. Unlike the Bohr Model, it explicitly accounts for electron-electron interactions and relativistic effects.
Schrodinger's Equation: A Glimpse into the Quantum World
The Schrodinger equation is a mathematical equation that describes the behavior of quantum mechanical systems, including atoms and molecules. Solving the Schrodinger equation for a given atom provides information about the allowed energy states and the corresponding wave functions, which describe the probability of finding an electron at a particular point in space.
While solving the Schrodinger equation for multi-electron atoms like sodium is complex, approximation methods exist to obtain highly accurate results. These calculations have provided detailed insights into the electronic structure of sodium.
Atomic Orbitals: Beyond Circular Paths
Instead of the well-defined orbits of the Bohr Model, quantum mechanics describes electrons as occupying atomic orbitals. These orbitals are three-dimensional regions of space around the nucleus. They represent the probability of finding an electron in that region.
Atomic orbitals have different shapes and energy levels, designated by quantum numbers. These numbers dictate the size, shape, and spatial orientation of the orbital. The familiar s, p, and d orbitals are examples of atomic orbitals with distinct shapes and energy levels. They provide a much more accurate representation of the electron distribution in sodium.
Video: Sodium Bohr Model: Unlocking Atomic Secrets!
FAQs: Sodium Bohr Model
What is the sodium Bohr model and what does it show?
The sodium Bohr model is a simplified representation of a sodium atom. It depicts the atom's nucleus surrounded by electrons orbiting in distinct energy levels, or shells. It helps visualize electron arrangement and energy levels, providing insight into sodium's chemical behavior.
How does the sodium Bohr model differ from the modern atomic model?
The Bohr model, including the sodium Bohr model, is a simplified concept. The modern atomic model uses quantum mechanics to describe electron behavior in orbitals, which are regions of probability, not fixed orbits. The sodium Bohr model is useful for basic understanding, but the quantum model offers a more accurate depiction.
What information about sodium can you gather from its Bohr model?
By examining the sodium Bohr model, you can see sodium has 11 electrons. You can also see it has one valence electron in its outermost shell. This single valence electron explains why sodium is highly reactive and readily forms positive ions.
How does the single valence electron in the sodium Bohr model relate to its reactivity?
Sodium's Bohr model shows only one electron in its outermost shell. To achieve a stable, full outer shell, sodium readily loses this electron. This ease of electron loss makes sodium a highly reactive metal, quickly forming ionic bonds with elements like chlorine.