Proton Charges: A Simple Guide to Atomic Structure
At the heart of every atom lies the nucleus, a dense region where protons reside and dictate the elemental identity, a fundamental principle explored deeply in chemistry. Understanding protons charges is essential for comprehending the behavior of elements on the periodic table, a cornerstone of Dmitri Mendeleev's groundbreaking work. These positively charged particles balance the negatively charged electrons orbiting the nucleus, a concept central to the Bohr model of the atom, and help to give structure to an atom.
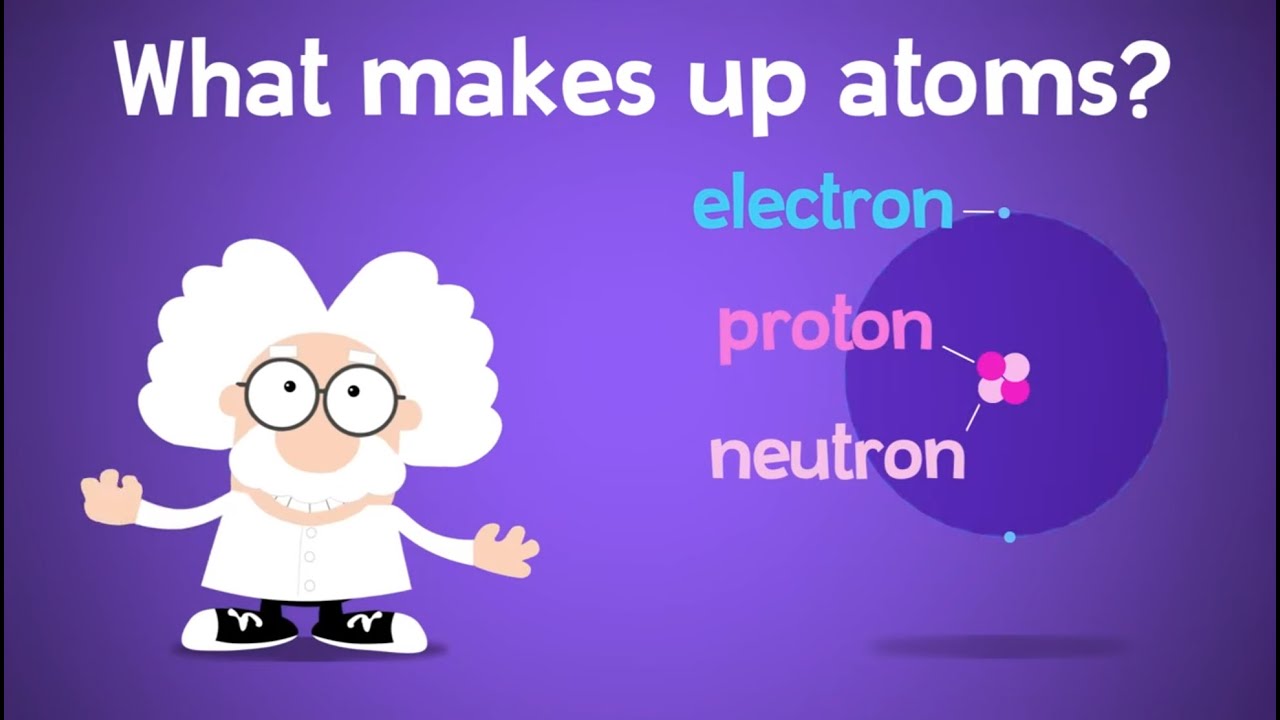
Image taken from the YouTube channel Tidlybit , from the video titled What's Inside an Atom? Protons, Electrons, and Neutrons! .
Unveiling the Mighty Proton: The Heart of Matter
At the heart of every atom, residing within the nucleus, lies the proton – a fundamental particle that dictates the very essence of the elements around us.
Understanding the proton is essential to unlocking the secrets of chemistry, physics, and the world as we know it.
Why Protons Matter: Defining Elements and Their Behavior
Protons are not just building blocks; they are the architects of the periodic table.
The number of protons in an atom's nucleus, known as the atomic number, uniquely identifies each element.
Change the number of protons, and you change the element itself! This pivotal role is the bedrock of understanding chemical behavior.
Protons and Atomic Identity
Hydrogen, with its single proton, is fundamentally different from helium, which boasts two.
This difference is not arbitrary; it defines their chemical properties and how they interact with other elements.
The atomic number, therefore, is more than just a number; it's the element's identity card.
What is a Proton? Core Attributes Explained
So, what exactly is a proton?
It's a subatomic particle carrying a positive electric charge.
This charge is equal in magnitude but opposite in sign to the charge of an electron.
Its mass is approximately 1 atomic mass unit (amu) or Dalton (Da), a standard unit for measuring atomic masses.
Diving into Charge
The proton's positive charge is considered the fundamental unit of positive charge.
It's often denoted as +e, where 'e' represents the elementary charge, approximately 1.602 x 10-19 Coulombs.
This fundamental charge governs the interactions between atoms and molecules.
Protons vs. Electrons: A Mass Disparity
While protons and electrons carry equal but opposite charges, their masses are drastically different.
A proton is approximately 1836 times more massive than an electron.
This mass difference is crucial for understanding the atom's structure.
Almost all of the atom's mass resides in the nucleus, concentrated in the protons and neutrons.
A Glimpse into the Past: Early Atomic Theories
The journey to discovering the proton was a gradual process, marked by evolving atomic theories.
Early models, like Dalton's atomic theory, laid the groundwork by suggesting that all matter is composed of indivisible atoms.
However, these models couldn't explain phenomena like electrical conductivity or the existence of different types of atoms.
The Need for a Nuclear Model
As experimental evidence accumulated, it became clear that the atom wasn't indivisible after all.
Scientists realized that there must be positively charged particles within the atom to balance the negatively charged electrons.
This realization paved the way for the development of the nuclear model, which revolutionized our understanding of atomic structure.
The Pioneers: Uncovering the Proton's Existence
The discovery of the proton wasn't a sudden flash of insight but rather the culmination of years of dedicated research, ingenious experiments, and evolving atomic theories. Let's explore the contributions of the key scientists who painstakingly pieced together the puzzle of the atom and revealed the existence of this fundamental particle.
Rutherford's Revolutionary Gold Foil Experiment
Ernest Rutherford's Gold Foil Experiment is arguably one of the most significant experiments in the history of atomic physics.
Rutherford and his team bombarded a thin gold foil with alpha particles (helium nuclei).
According to the then-accepted "plum pudding" model of the atom (where electrons were scattered throughout a positively charged "pudding"), the alpha particles should have passed through the foil with only minor deflections.
However, what they observed was astonishing:
Most alpha particles did pass straight through, but a small fraction were deflected at large angles, and some even bounced directly back!
A New Model of the Atom Emerges
This unexpected result led Rutherford to propose a radical new model of the atom.
He concluded that the atom's positive charge and most of its mass were concentrated in a tiny, dense core – the nucleus.
This meant that the atom was mostly empty space, explaining why most alpha particles passed through undeflected.
The rare, large-angle deflections were caused by the alpha particles encountering the concentrated positive charge of the nucleus.
This groundbreaking experiment effectively dismantled the plum pudding model and gave birth to the nuclear model of the atom, a cornerstone of modern physics.
Chadwick: Completing the Nuclear Picture with the Neutron
While Rutherford's experiment revealed the existence of the positively charged nucleus, it didn't fully explain the mass of atoms.
The atomic weight of an element was roughly double the number of protons.
This discrepancy led scientists to believe that there must be another particle within the nucleus, contributing to its mass but without any electric charge.
James Chadwick, a student of Rutherford, took on this challenge.
The Discovery of the Neutron
In 1932, Chadwick conducted a series of experiments in which he bombarded beryllium with alpha particles, producing a previously unknown radiation.
Through careful analysis, he determined that this radiation consisted of neutral particles with a mass similar to that of the proton.
He had discovered the neutron.
Chadwick's discovery completed the picture of the nucleus, explaining the mass discrepancy and providing a crucial piece of the atomic puzzle.
The discovery of the neutron also helped explain the existence of isotopes, atoms of the same element with different numbers of neutrons.
Precursors to Discovery: Thomson and Goldstein Lay the Groundwork
While Rutherford and Chadwick are credited with discovering the nucleus and the neutron respectively, their work built upon the foundation laid by earlier scientists.
J.J. Thomson's discovery of the electron in 1897 was a crucial step in understanding the structure of the atom and the nature of electric charge.
Thomson and the Electron
Thomson demonstrated that cathode rays were streams of negatively charged particles, which he called "corpuscles" (later named electrons).
This discovery not only identified a fundamental constituent of matter but also raised the question of how these negatively charged particles were balanced within the atom to create electrical neutrality.
Goldstein and Canal Rays
Prior to Thomson, Eugen Goldstein's experiments with cathode ray tubes led to the observation of "canal rays" (also known as anode rays or positive rays).
Goldstein used a perforated cathode, and noticed a faint glow originating from the holes ("canals") on the back side of the cathode when high voltage was applied.
These rays were deflected by magnetic fields, indicating they were composed of charged particles.
While Goldstein didn't identify them as protons, his work with canal rays demonstrated the existence of positively charged particles that were later understood to be positive ions formed when electrons were stripped from gas atoms in the tube.
This paved the way for later experiments that would ultimately identify the proton as a fundamental particle.
Protons and Atomic Identity: The Atomic Number
Now that we've explored the discovery and fundamental nature of protons, it's time to understand their crucial role in defining the very essence of an element. The number of protons within an atom's nucleus isn't just a random quantity; it's the atomic number, a defining characteristic that dictates an element's identity and its chemical behavior. Let's dive into how this single number unlocks the secrets of the periodic table.
Atomic Number (Z): The Definitive Elemental Identifier
Think of the atomic number as an element's unique fingerprint. It's the single most important piece of information because it tells us exactly what element we're dealing with. Each element on the periodic table is distinguished by its unique atomic number.
For example, every atom with one proton is hydrogen (atomic number 1), every atom with six protons is carbon (atomic number 6), and every atom with 79 protons is gold (atomic number 79).
The Power of Proton Transformation
The atomic number is immutable unless a nuclear reaction occurs. Changing the number of protons fundamentally transforms one element into another. This is the basis of nuclear transmutation, where elements can be converted into different elements through processes like nuclear fission or fusion.
For instance, bombarding nitrogen atoms with alpha particles (two protons and two neutrons) can result in the formation of oxygen atoms. This is because the nitrogen atom gains a proton, effectively changing its atomic number from 7 to 8, thus becoming oxygen.
The Periodic Table Connection
The periodic table is organized in order of increasing atomic number. This arrangement highlights the periodic trends in chemical properties that arise from the electronic structure of atoms, which is, in turn, dictated by the number of protons in the nucleus.
Elements in the same group (vertical column) share similar chemical properties because they have the same number of valence electrons (electrons in the outermost shell), a consequence of their similar electronic configurations arising from the number of protons.
Mass Number (A): The Sum of Protons and Neutrons
While the atomic number defines the type of element, the mass number provides information about the total number of nucleons (protons and neutrons) within the nucleus. The mass number (symbol A) is simply the sum of protons and neutrons.
A = Z + N
where:
- A = Mass Number
- Z = Atomic Number (number of protons)
- N = Number of neutrons
The mass number is a whole number, unlike the atomic mass (which is a weighted average of the masses of all naturally occurring isotopes).
Isotopes and Mass Number Variation
The mass number can vary for different atoms of the same element. These variations are called isotopes. Because isotopes have the same number of protons (same atomic number) but different numbers of neutrons, they have different mass numbers.
For example, carbon-12 (¹²C) has 6 protons and 6 neutrons (mass number 12), while carbon-14 (¹⁴C) has 6 protons and 8 neutrons (mass number 14). Both are carbon because they both have 6 protons, but they are different isotopes.
Isotopes: Variations on an Atomic Theme
As touched on previously, isotopes are atoms of the same element (same atomic number, Z) that have different numbers of neutrons (N), leading to different mass numbers (A).
Isotopes are typically denoted by the element symbol with the mass number as a superscript to the left (e.g., ¹⁴C) or by the element name followed by the mass number (e.g., carbon-14).
The Impact on Average Atomic Mass
Because elements often exist as a mixture of isotopes, the atomic mass listed on the periodic table is a weighted average of the masses of all the naturally occurring isotopes of that element, taking into account their relative abundance. This explains why atomic masses are not always whole numbers.
Examples and Applications of Isotopes
Isotopes have a wide range of applications in various fields:
-
Carbon-14 dating: This is a radiometric dating technique that uses the decay of carbon-14 to estimate the age of organic materials up to around 50,000 years old.
-
Medical imaging: Radioactive isotopes like iodine-131 are used in medical imaging and treatment to diagnose and treat thyroid disorders.
-
Nuclear power: Uranium-235 is a fissile isotope used as fuel in nuclear reactors.
-
Scientific research: Stable isotopes (non-radioactive) are used as tracers in chemical and biological research to study reaction mechanisms and metabolic pathways.
Charge and Interactions: Forces at Play Within the Atom
Having established the fundamental role of protons in determining atomic identity, we now turn our attention to the dynamic forces and interactions that govern their behavior within the atom. These forces are not merely static; they are the very engine that drives atomic structure and stability, orchestrating a delicate dance of attraction and repulsion.
Electrical Neutrality: A Balancing Act
Atoms, in their natural state, exhibit electrical neutrality, a state of equilibrium where the total positive charge perfectly cancels out the total negative charge. This is achieved through a simple yet elegant mechanism: an equal number of protons and electrons. Each proton carries a single positive charge (+1e), while each electron carries a single negative charge (-1e). This balance is critical for the overall stability of matter as we know it.
Imagine a tug-of-war where both sides are pulling with equal force. The rope remains stationary. Similarly, in a neutral atom, the positive pull of the protons is precisely balanced by the negative pull of the electrons.
Ions: When the Balance Tips
However, this perfect balance is not always maintained. Atoms can gain or lose electrons, leading to the formation of ions. When an atom loses one or more electrons, it becomes positively charged and is called a cation. Conversely, when an atom gains one or more electrons, it becomes negatively charged and is called an anion.
The charge of an ion is directly determined by the difference between the number of protons and electrons. For example, a sodium atom (Na) with 11 protons and 11 electrons is neutral. If it loses one electron, it becomes a sodium ion (Na+) with a +1 charge, having 11 protons but only 10 electrons.
Electromagnetic Force: The Attraction That Binds
One of the fundamental forces of nature, the electromagnetic force, plays a crucial role in holding the atom together. This force governs the attraction between oppositely charged particles. In the context of the atom, it's the electromagnetic force that draws negatively charged electrons towards the positively charged nucleus, a marriage made in atomic heaven.
The electromagnetic force is responsible for holding the electrons in their orbits around the nucleus. The strength of the attraction depends on the magnitude of the charges and the distance between them. The closer the electron is to the nucleus, the stronger the attraction.
Electrostatic Repulsion: Protons Pushing Away
While the electromagnetic force is busy attracting electrons to the nucleus, another force is at play within the nucleus itself: electrostatic repulsion. Since protons all carry positive charges, they naturally repel each other.
This repulsion is a significant factor in determining the stability of the nucleus. One might wonder, if protons are repelling each other, why doesn't the nucleus simply fly apart? The answer lies in another, even stronger force.
Strong Nuclear Force: The Ultimate Glue
To counteract the electrostatic repulsion between protons, nature provides an even more powerful force: the strong nuclear force. This force, acting at very short distances, overcomes the electrostatic repulsion and binds protons and neutrons together within the nucleus. It is aptly named "strong" because it is indeed the strongest of the four fundamental forces of nature.
The strong nuclear force is essentially the glue that holds the nucleus together. It acts equally between protons and neutrons, ensuring the stability of the atomic nucleus. Without the strong nuclear force, all atoms with more than one proton would be unstable, and matter as we know it would not exist.
Charge Quantization: Discrete Units of Electrical Charge
Finally, it's important to understand that electric charge is quantized. This means that charge doesn't come in just any arbitrary amount, but in discrete units. The smallest unit of charge is the elementary charge, denoted as 'e', which is the magnitude of the charge carried by a single proton or electron (approximately 1.602 x 10^-19 Coulombs).
Any measurable electric charge will always be an integer multiple of this elementary charge. You can't have half a proton's charge; you can only have whole numbers of elementary charges. This quantization of charge is a fundamental principle of physics with far-reaching consequences.
Measuring Protons: Atomic Mass and Mass Spectrometry
Having established the fundamental role of protons in determining atomic identity, we now turn our attention to the methods scientists use to measure and quantify these subatomic particles. Understanding these measurement techniques is crucial for grasping how we've come to know so much about the atomic world.
This section will explore the atomic mass unit (amu) and the Dalton (Da) – the tiny units of mass used in this realm – and the powerful technique of mass spectrometry.
Atomic Mass Unit (amu) / Dalton (Da): The Tiny Unit of Mass
When dealing with the incredibly small masses of atoms and subatomic particles like protons, everyday units like grams or kilograms become unwieldy. That's where the atomic mass unit (amu), also known as the Dalton (Da), comes in.
It provides a convenient scale for expressing these minuscule masses. Think of it as the "atomic ruler" for measuring mass.
Defining the amu/Dalton
The amu/Dalton is defined based on the mass of a neutral carbon-12 ((^{12}C)) atom. By international agreement, one amu/Dalton is equal to 1/12 of the mass of a single, isolated carbon-12 atom in its ground state. This definition provides a precise and reproducible standard.
The Proton's Mass in amu/Daltons
So, what's the mass of a proton in these units? A proton has a mass of approximately 1.00728 amu or 1.00728 Da.
For most practical purposes, it's often rounded to just 1 amu or 1 Da. This slight difference is important in high-precision calculations.
It is good to keep it in mind for understanding nuclear binding energies.
Mass Spectrometry: Separating Atoms by Mass and Charge
How do scientists actually measure the masses of individual atoms and their isotopes? The answer lies in a sophisticated technique called mass spectrometry.
Mass spectrometry is an analytical technique used to identify and quantify the different isotopes of elements, as well as molecules, based on their mass-to-charge ratio. It's like a super-sensitive scale that can weigh individual atoms and molecules with incredible accuracy.
How Mass Spectrometry Works: A Step-by-Step Overview
The process of mass spectrometry generally involves four key steps:
-
Ionization: The sample is first ionized, meaning that atoms or molecules are given an electrical charge (either positive or negative). This is crucial because it allows them to be manipulated by electric and magnetic fields. Often, electrons are knocked off the atoms to create positive ions.
-
Acceleration: The ions are then accelerated through an electric field. This gives all the ions the same kinetic energy.
-
Deflection: Next, the ions pass through a magnetic field. The amount of deflection depends on the ion's mass-to-charge ratio (m/z). Lighter ions and ions with a higher charge are deflected more.
-
Detection: Finally, the ions are detected. The detector measures the abundance of each ion at each m/z value. This data is then used to generate a mass spectrum, which is a plot of ion abundance versus m/z.
By analyzing the mass spectrum, scientists can determine the isotopic composition of a sample, identify unknown compounds, and even study the structure of molecules. Mass spectrometry truly unveils the atomic makeup of matter.
The Proton in Context: Atomic Models and Laboratories
Having established the fundamental role of protons in determining atomic identity, we now turn our attention to the individuals and institutions that shaped our modern understanding of these particles. Exploring these historical contexts adds further depth to the discovery and conceptualization of the proton within atomic structure.
Niels Bohr and the Dawn of Quantum Mechanics
Niels Bohr, a towering figure in 20th-century physics, played a pivotal role in integrating the concept of the nucleus, replete with protons and neutrons, into a revolutionary atomic model. Bohr's model, developed in the early 1910s, represented a significant departure from classical physics by introducing the concept of quantized electron orbits.
Instead of orbiting the nucleus in any arbitrary path, Bohr postulated that electrons could only exist in specific, discrete energy levels, like rungs on a ladder. This groundbreaking idea was a critical step toward explaining the behavior of atoms and the light they emit.
Explaining Atomic Spectra
One of the most compelling pieces of evidence supporting Bohr's model came from its ability to accurately predict and explain atomic spectra. When atoms are excited, they emit light at specific wavelengths, creating a unique spectral fingerprint for each element.
Bohr's model elegantly explained these spectral lines as arising from electrons transitioning between energy levels, releasing or absorbing photons of light with specific energies. This achievement solidified the significance of Bohr's model and cemented his place as a central figure in the development of quantum mechanics.
The Cavendish Laboratory: A Crucible of Discovery
The Cavendish Laboratory at the University of Cambridge holds a special place in the history of physics. Throughout the late 19th and early 20th centuries, it served as a vibrant hub for groundbreaking research in electromagnetism and atomic physics.
Under the leadership of visionary directors like J.J. Thomson and Ernest Rutherford, the Cavendish fostered a culture of experimentation and theoretical innovation that led to a series of remarkable discoveries. It was within these hallowed halls that the electron was discovered by Thomson, revolutionizing our concept of the atom.
Moreover, Rutherford conducted his transformative Gold Foil experiment, which unveiled the nuclear structure of the atom and paved the way for the identification of the proton. Later, James Chadwick, also working at the Cavendish, discovered the neutron, completing our understanding of the fundamental constituents of the atomic nucleus.
The Cavendish Laboratory exemplifies how a dedicated scientific community, with visionary leaders and cutting-edge facilities, can propel our understanding of the universe forward. It remains a testament to the power of collaborative and curiosity-driven research.
Video: Proton Charges: A Simple Guide to Atomic Structure
Frequently Asked Questions
What is the role of protons in an atom?
Protons, along with neutrons, reside in the nucleus of an atom. The number of protons determines the element's identity. Each element has a specific number of protons; changing this number changes the element. The positive protons charges are crucial for balancing the negatively charged electrons.
How do protons affect an atom's charge?
Atoms are normally neutral because the number of positively charged protons equals the number of negatively charged electrons. If an atom gains or loses electrons, it becomes an ion with a net charge. It is the balance with the protons charges that determine whether the atom is neutral, positive, or negative.
Why are protons so important in chemical reactions?
While electrons are primarily involved in forming chemical bonds, the number of protons dictates the atom's identity and its inherent chemical properties. This influences how it will interact with other atoms. The existence of positive protons charges ensures the correct number of electrons are present.
Do protons ever change during normal chemical reactions?
Generally, no. The number of protons in an atom's nucleus defines the element. Chemical reactions involve the sharing or transfer of electrons. Changes to the number of protons would mean changing the element itself, which requires nuclear reactions. Stable protons charges preserve the element.
So, there you have it! Hopefully, this clears up any confusion you might have had about protons, neutrons, and electrons, especially regarding those all-important positive protons charges. Now you can confidently explain the basics of atomic structure at your next trivia night!