Unlock the Potassium Bohr Model's Secrets Now!
The Bohr model, a cornerstone of early atomic theory, provides a simplified yet powerful framework for understanding electron behavior. Specifically, the potassium bohr model demonstrates unique electron configurations. Analyzing this requires understanding the electron configuration and the principles of quantum mechanics. The insights gleaned from studying the potassium bohr model can illuminate more complex atomic structures, like those explored by scientists at institutions like the Niels Bohr Institute.
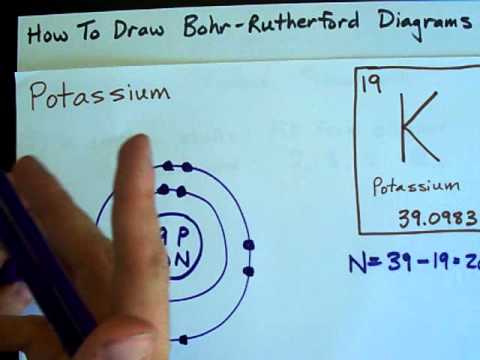
Image taken from the YouTube channel chemistNATE , from the video titled How to Draw Bohr-Rutherford Diagrams - Potassium .
Potassium, represented by the symbol K from the Latin "kalium," is far more than just another element on the periodic table. It is a critical component of life itself, playing an indispensable role in processes ranging from nerve impulse transmission to maintaining fluid balance within our cells.
But here's a surprising fact: potassium's radioactivity, stemming from the isotope Potassium-40, makes it one of the primary sources of natural radioactivity within the human body.
It's a silent partner in our existence, constantly at work to keep us functioning.
This article aims to demystify the seemingly complex world of atomic structure by exploring the Bohr Model through the lens of potassium. We will unveil the secrets of its atomic configuration and understand how these contribute to potassium's unique behavior.
Potassium (K): An Essential Element
Potassium is an essential mineral that our bodies need to function correctly.
It is categorized as an alkali metal, sharing properties with elements like lithium and sodium. These properties dictate how it interacts with other substances.
Its significance extends beyond human biology, impacting agriculture as a key component of fertilizers and playing a role in various industrial processes.
The Potassium Hook: A Radioactive Spark Within
While not typically associated with radioactivity, potassium possesses a naturally occurring radioactive isotope: potassium-40. This isotope makes up a small fraction of all potassium, but it's significant.
It contributes to the background radiation we all experience daily.
This unexpected property serves as a fascinating entry point into the more profound aspects of its atomic structure. It underscores the importance of understanding the behavior of even the most seemingly simple elements.
Thesis: Demystifying Potassium Through the Bohr Model
This exploration dives into the application of the Bohr Model to potassium. The Bohr Model provides a simplified, yet valuable framework. The Bohr Model helps us understand the arrangement of electrons within potassium's atomic structure.
By utilizing this model, we'll uncover the relationship between its electron configuration and its chemical behavior.
Ultimately, we will understand how the Bohr Model provides essential insights into potassium's reactivity and its place in the world around us.
Potassium, with its intriguing radioactive isotope, offers a glimpse into the complex world of the atom. But to truly understand potassium's unique characteristics, we must first lay the groundwork by exploring the model that revolutionized our understanding of atomic structure: the Bohr Model.
Unveiling the Bohr Model: A Foundation of Atomic Understanding
The Bohr Model, while superseded by more advanced theories, remains a cornerstone of atomic theory education. Its simplicity and intuitive nature make it an invaluable tool for grasping the fundamental principles governing atomic behavior.
The Core Principles: Quantized Energy Levels
At the heart of the Bohr Model lies the concept of quantized energy levels. This revolutionary idea postulates that electrons can only exist in specific, discrete orbits around the nucleus, much like planets orbiting a star at fixed distances.
Electrons cannot occupy the space between these orbits.
Each orbit corresponds to a specific energy level, with electrons in orbits closer to the nucleus possessing lower energy than those in orbits farther away. These energy levels are often visualized as concentric shells, each capable of holding a specific number of electrons.
This quantization of energy is a radical departure from classical physics, which allows for a continuous range of energy values.
Niels Bohr: A Pioneer of Atomic Theory
The Bohr Model is inextricably linked to its creator, Niels Bohr, a Danish physicist who made groundbreaking contributions to our understanding of the atom.
In 1913, Bohr proposed his model, incorporating concepts from quantum theory to address the shortcomings of earlier atomic models, such as the Rutherford model.
Bohr's model successfully explained the discrete spectral lines observed in hydrogen, a feat that earned him the Nobel Prize in Physics in 1922. His work paved the way for the development of modern quantum mechanics.
Limitations and Legacy
Despite its successes, the Bohr Model is not without its limitations. It accurately predicts the behavior of hydrogen, which has only one electron, but it struggles to explain the spectra of more complex atoms.
The model also fails to account for the wave-particle duality of electrons and does not accurately describe the shapes of atomic orbitals.
Furthermore, the Bohr model does not adhere to Heisenberg's Uncertainty Principle.
Quantum mechanics provides a more complete and accurate description of atomic structure and behavior.
However, the Bohr Model should not be dismissed as obsolete.
Its simplicity and visualizability make it an excellent pedagogical tool for introducing the fundamental concepts of atomic structure, such as quantized energy levels, electron shells, and electron transitions. It serves as a crucial stepping stone toward understanding the more complex and abstract concepts of quantum mechanics.
Deconstructing Potassium: Atomic Structure and Electron Configuration
Having explored the fundamental principles of the Bohr Model, we can now apply this knowledge to unravel the structure of potassium itself. Understanding the arrangement of protons, neutrons, and electrons within a potassium atom is crucial to grasping its chemical behavior.
Unveiling Potassium's Atomic Nucleus
At the heart of every potassium atom lies the nucleus, a densely packed core composed of protons and neutrons.
Potassium (K) has an atomic number of 19, which signifies that each potassium atom possesses 19 protons. These positively charged particles define the element's identity.
The number of neutrons, however, can vary, leading to different isotopes of potassium. The most common isotope, potassium-39 (³⁹K), contains 20 neutrons.
Therefore, its nucleus contains 19 protons and 20 neutrons. This gives it an atomic mass of approximately 39 atomic mass units (amu).
The Electron Cloud: Orbitals and Energy Levels
Surrounding the nucleus is the electron cloud, a region where electrons are most likely to be found.
In a neutral potassium atom, the number of electrons is equal to the number of protons. Therefore, potassium has 19 electrons.
These electrons are not randomly distributed but occupy specific energy levels or electron shells, as described by the Bohr Model.
Deciphering Electron Configuration
Electron configuration describes the arrangement of electrons within these energy levels and sublevels.
For potassium, the electron configuration is: 1s² 2s² 2p⁶ 3s² 3p⁶ 4s¹.
Let's break down this notation:
- 1s²: The first energy level (n=1) has one s orbital, which contains two electrons.
- 2s² 2p⁶: The second energy level (n=2) has one s orbital (2s²) and three p orbitals (2p⁶), accommodating a total of eight electrons.
- 3s² 3p⁶: The third energy level (n=3) follows a similar pattern, with two electrons in the s orbital (3s²) and six electrons in the p orbitals (3p⁶), again totaling eight electrons.
- 4s¹: Finally, the fourth energy level (n=4) contains only one electron in its s orbital (4s¹).
The order in which these energy levels are filled follows the Aufbau principle, which states that electrons first occupy the lowest energy levels available.
This filling order is crucial for predicting an element's chemical properties.
The Principal Quantum Number (n): Defining Electron Shells
The principal quantum number, denoted as n, is a fundamental concept in understanding atomic structure. It defines the energy level or electron shell that an electron occupies.
n can be any positive integer (1, 2, 3, and so on), with higher values indicating higher energy levels and greater distances from the nucleus.
In potassium's electron configuration, the numbers 1, 2, 3, and 4 represent the principal quantum numbers for each electron shell.
The first shell (n=1) is closest to the nucleus, followed by the second (n=2), third (n=3), and fourth (n=4) shells. Each shell can hold a maximum number of electrons, determined by the formula 2n².
Thus the first shell can hold 2 electrons, the second shell can hold 8 electrons, the third shell can hold 18 electrons, and so on. Potassium does not completely fill its third shell, instead placing its 19th electron in the fourth shell.
Having established potassium's atomic structure and meticulously mapped its electron configuration, we now turn our attention to the element's "personality"—its propensity to react with other substances. This reactivity stems from a single, crucial aspect of its electron arrangement.
Valence Electrons: Potassium's Reactive Personality Explained
The key to understanding potassium's reactivity lies in its valence electrons. These are the electrons residing in the outermost electron shell of an atom. They are the electrons most involved in chemical bonding.
The Lone Electron
Consider potassium's electron configuration: 1s² 2s² 2p⁶ 3s² 3p⁶ 4s¹. Notice that the fourth energy level (n=4) contains only one electron, occupying the 4s orbital. This single electron is potassium's valence electron.
This lone electron is rather far from the positively charged nucleus. It's shielded by the other 18 electrons. Thus, it's relatively easy to remove compared to the inner electrons.
Alkali Metal Behavior
Potassium belongs to Group 1 of the periodic table, also known as the alkali metals. Alkali metals are renowned for their high reactivity. This is directly attributable to their electron configurations. Each alkali metal possesses only one valence electron.
They are all eager to lose this single electron to achieve a more stable electron configuration, resembling that of a noble gas.
Achieving Stability Through Ionization
When potassium loses its valence electron, it forms a positively charged ion, K⁺. This ion has the same electron configuration as argon (Ar), a noble gas. Noble gases are exceptionally stable due to their full outermost electron shells.
The process of losing an electron is known as ionization. Potassium readily undergoes ionization, readily giving away its valence electron to form chemical bonds with other elements.
Reactivity in Action
The ease with which potassium loses its valence electron explains its high reactivity. For example, potassium reacts vigorously with water, producing hydrogen gas and potassium hydroxide.
2K(s) + 2H₂O(l) → 2KOH(aq) + H₂(g)
This reaction is highly exothermic, releasing a significant amount of heat. This further demonstrates potassium's eagerness to achieve a more stable electron configuration by shedding its lone valence electron. The released energy during the reaction is due to the formation of strong bonds between potassium and hydroxide.
Connection to Electron Configuration
Potassium's electron configuration is therefore not merely an abstract notation. It is a roadmap to understanding its chemical behavior. The 4s¹ configuration explains the existence of one loosely bound valence electron. This explains its tendency to readily lose that electron. This loss ultimately drives its high reactivity as an alkali metal.
Understanding the electron configuration allows us to predict and explain its interactions with other elements.
Having established potassium's atomic structure and meticulously mapped its electron configuration, we now turn our attention to the element's "personality"—its propensity to react with other substances. This reactivity stems from a single, crucial aspect of its electron arrangement.
Potassium's Light Show: Linking the Bohr Model to Emission Spectrum
Beyond its eagerness to form chemical bonds, potassium showcases its atomic identity through a fascinating phenomenon: its atomic emission spectrum. When potassium atoms are excited, they emit light, but not just any light. It emits light at very specific wavelengths, creating a unique "fingerprint" of spectral lines.
This light show provides a powerful visual demonstration of the principles of the Bohr model and reveals the intricate relationship between electron energy levels and light emission.
Understanding Atomic Emission Spectra
Every element possesses a unique atomic emission spectrum. When an element is heated or subjected to electrical discharge, its electrons absorb energy and jump to higher energy levels.
These higher energy levels are unstable.
The excited electrons almost immediately fall back down to lower energy levels, releasing the excess energy in the form of photons of light.
The energy of each photon corresponds to the difference in energy between the two energy levels involved in the transition. Because these energy level differences are quantized (meaning they can only take on specific, discrete values), the emitted light has only specific wavelengths.
The Role of Potassium's Valence Electron
Potassium's single valence electron is the key player in its emission spectrum. It's this lone electron that is most easily excited to higher energy levels.
When this electron falls back to its ground state (the lowest energy level), or to intermediate energy levels, it emits photons of specific wavelengths characteristic of potassium.
These wavelengths appear as distinct lines in the emission spectrum.
The vibrant violet color often observed in potassium flame tests is a result of these specific electron transitions and the emitted light's corresponding wavelengths.
Ground State and Excited State: A Tale of Two Energy Levels
An electron in its normal, unexcited state resides in the ground state, the lowest possible energy level for that electron.
When an electron absorbs energy, it transitions to a higher energy level, known as the excited state.
The excited state is inherently unstable. An electron will spontaneously return to a lower energy level, releasing the absorbed energy as a photon.
The greater the energy difference between the excited state and the final energy level, the higher the energy (and shorter the wavelength) of the emitted photon.
This relationship between energy levels and emitted light is precisely what the Bohr model helps to explain and visualize. The model's concept of quantized energy levels provides a framework for understanding why elements emit light at specific, predictable wavelengths, giving rise to their unique atomic emission spectra.
Having illuminated how potassium's electron configuration dictates its light emission, it's time to zoom out and consider its broader context within the periodic table. Potassium doesn't exist in isolation. Its behavior and characteristics are deeply intertwined with its placement in the alkali metal family, a group of elements that share a common thread of reactivity and electron configuration.
Potassium's Place: The Alkali Metal Family
Potassium's identity is not solely defined by its unique atomic properties; it's also shaped by its membership in the alkali metal family. This group, residing in Group 1 of the periodic table, shares characteristic behaviors and electron configurations that dictate their reactivity.
Alkali Metals: A Family Portrait
The alkali metals—Lithium (Li), Sodium (Na), Potassium (K), Rubidium (Rb), Cesium (Cs), and Francium (Fr)—are renowned for their extreme reactivity. This shared trait stems from a unifying factor: each possesses a single valence electron in its outermost electron shell.
This lone electron is loosely bound and readily donated, making these elements eager to form positive ions (+1 charge) and readily bond with other elements.
The Periodic Table: Location, Location, Location
Potassium's position in Group 1 immediately signals its alkali metal nature. As you move down the group, reactivity generally increases. This trend is directly linked to the distance between the nucleus and the valence electron.
As you descend the group, the valence electron occupies higher energy levels, further from the nucleus. This increased distance weakens the attraction between the nucleus and the valence electron.
With decreased attraction, this valence electron becomes easier to remove, resulting in higher reactivity.
Electron Configuration and Reactivity: A Family Affair
While all alkali metals share the single valence electron characteristic, their electron configurations differ in the number of filled inner shells.
For instance:
- Lithium (Li): [He] 2s¹
- Sodium (Na): [Ne] 3s¹
- Potassium (K): [Ar] 4s¹
The noble gas core ([He], [Ne], [Ar]) represents the filled inner electron shells. Each alkali metal simply adds one electron to the 's' orbital of the next period.
This seemingly minor difference in the principal quantum number (n) of the valence electron has a profound impact on reactivity. Potassium, with its valence electron in the 4s orbital, is more reactive than sodium (3s) and lithium (2s) because its valence electron is farther from the nucleus and therefore more easily removed.
Comparing Potassium to Its Siblings: A Tale of Reactivity
Lithium, being the smallest alkali metal, exhibits some unique properties due to its high charge density. It's less reactive than sodium and potassium in many reactions.
Sodium, commonly known as table salt, is more reactive than lithium but less reactive than potassium.
Potassium reacts vigorously with water, generating heat and hydrogen gas. Rubidium and Cesium react even more violently, and Francium is so radioactive that it doesn't have many stable isotopes for observable reactions.
Potassium's intermediate position in the family provides a compelling case study for understanding the interplay between electron configuration, atomic size, and chemical reactivity. Its eagerness to shed its valence electron and achieve a stable electron configuration like that of argon solidifies its place as a quintessential alkali metal.
Video: Unlock the Potassium Bohr Model's Secrets Now!
Unlocking the Potassium Bohr Model: Your Questions Answered
Here are some frequently asked questions to help you better understand the intricacies of the Potassium Bohr Model.
What exactly is the Bohr model and how does it relate to potassium?
The Bohr model is a simplified model of the atom where electrons orbit the nucleus in specific energy levels or shells. In the potassium bohr model, these shells are populated with potassium's 19 electrons, following specific filling rules. It visualizes the electron arrangement for easier understanding.
What are the limitations of using the Bohr model to describe potassium?
The Bohr model is a simplified representation and doesn't fully account for the complex interactions between electrons, electron orbitals, and the nucleus. It is useful for basic visualization but does not reflect all properties of the actual potassium atom.
How does the electron configuration of potassium influence its chemical properties?
Potassium's electron configuration, specifically the single electron in its outermost shell, makes it highly reactive. This single electron is readily lost, forming a +1 ion and allowing potassium to easily bond with other elements. The potassium bohr model can help to visualize the lone electron in its outer shell.
What are some real-world applications related to understanding potassium's atomic structure?
Understanding potassium's atomic structure is vital in fields like fertilizer production (potassium is a key nutrient for plant growth), battery technology (potassium-ion batteries are being developed), and medicine (potassium balance is crucial for cell function). Knowledge of the potassium bohr model aids in these developments.