CPM Radiation: Safe Levels, Risks & Exposure
Counts Per Minute (CPM), a common unit of measurement, quantifies the rate of detected ionizing events, and is especially crucial in understanding CPM radiation levels. Organizations such as the International Atomic Energy Agency (IAEA) establish guidelines for acceptable CPM radiation thresholds, striving to minimize potential health risks. Instruments like Geiger counters are essential tools for accurately measuring CPM radiation in various environments. Evaluating exposure to CPM radiation is vital, given the potential risks, particularly when levels exceed established safety standards.
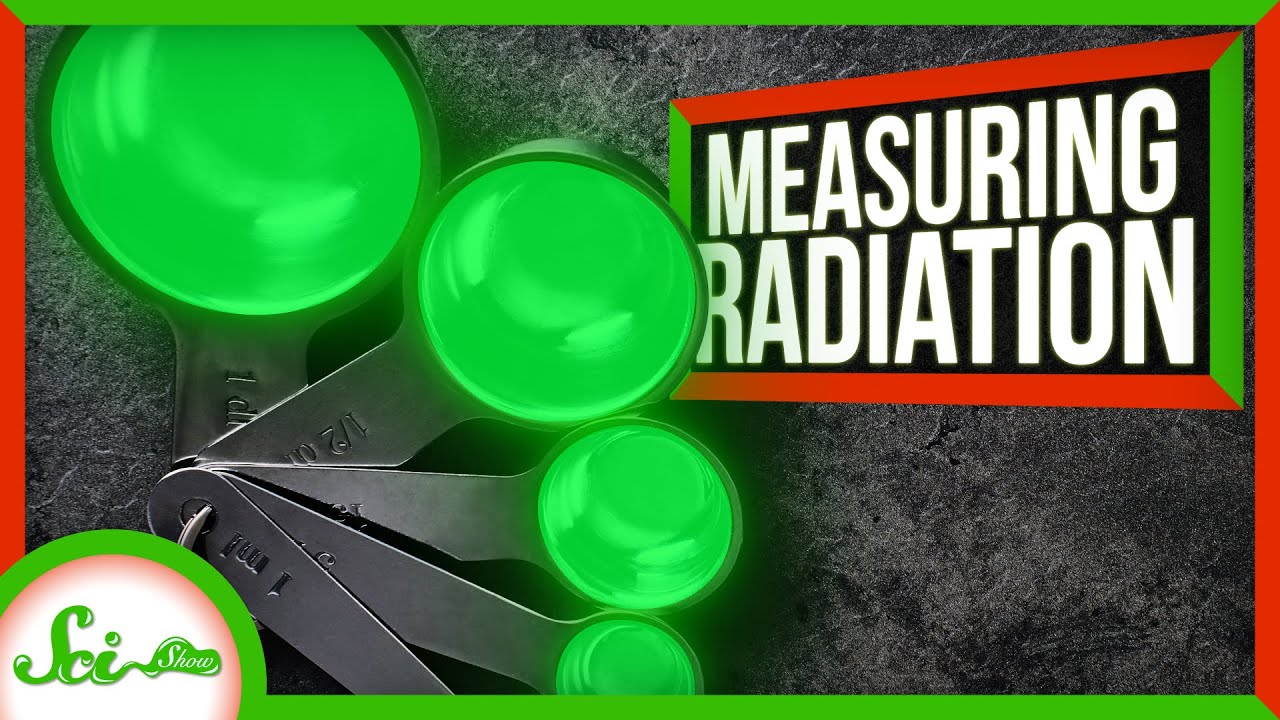
Image taken from the YouTube channel SciShow , from the video titled The Only Radiation Units You Need to Know .
Radiation: A Fundamental Overview
Radiation, a ubiquitous phenomenon permeating our universe, plays a pivotal role in shaping our world. From the life-sustaining energy of the sun to the transformative applications in medicine and industry, understanding radiation is crucial in our modern era.
This section will lay the groundwork for a deeper exploration, clarifying what radiation is, why its understanding is paramount, and the crucial distinctions between its various forms, particularly ionizing and non-ionizing radiation.
Defining Radiation: Energy in Transit
At its core, radiation is the emission or transmission of energy through space or a material medium. This energy can take many forms, including electromagnetic waves (like light and radio waves) and particulate radiation (like alpha and beta particles).
Fundamentally, it represents energy in transit, capable of interacting with matter in various ways. This interaction is what gives rise to both the benefits and potential hazards associated with radiation.
The Significance of Understanding Radiation
The implications of radiation extend across numerous critical sectors.
Medicine
In medicine, radiation is used in diagnostics, such as X-rays and CT scans, to visualize internal structures. Radiation therapy employs focused beams to target and destroy cancerous cells.
Nuclear Energy
Nuclear energy relies on controlled nuclear reactions, which release tremendous amounts of energy in the form of radiation. Understanding how to harness and contain this radiation is essential for safe and efficient power generation.
Environmental Monitoring
Environmental monitoring utilizes radiation detection to track pollutants, assess radioactive contamination, and ensure public safety. Monitoring radiation level is also critical in protecting humans and animals from harm.
Industrial Applications
Many industrial processes, from sterilization of medical equipment to gauging the thickness of materials, rely on radiation technologies. Accurate radiation measurement ensures quality control and worker safety.
Ionizing vs. Non-Ionizing Radiation: A Critical Distinction
The nature of radiation and its impact hinges on its ability to ionize atoms and molecules. This leads to the crucial distinction between ionizing and non-ionizing radiation.
Ionizing Radiation
Ionizing radiation carries enough energy to remove electrons from atoms, creating ions. This process can damage DNA and other biological molecules, potentially leading to adverse health effects.
Examples include alpha particles, beta particles, gamma rays, and X-rays. Due to their capacity to alter molecular structures, exposure to ionizing radiation requires careful management.
Non-Ionizing Radiation
Non-ionizing radiation, on the other hand, does not have sufficient energy to ionize atoms. While generally considered less harmful than ionizing radiation, high levels can still cause thermal effects.
Examples include radio waves, microwaves, infrared radiation, visible light, and ultraviolet (UV) radiation. For instance, prolonged exposure to UV radiation from the sun can cause sunburn and increase the risk of skin cancer, highlighting the need for moderation even with non-ionizing sources.
Core Concepts and Units of Measurement: Quantifying Radiation
Building upon the fundamental understanding of radiation, we now delve into the critical concepts and units that enable us to quantify and assess its presence and potential impact. Accurate measurement is paramount for informed decision-making in radiation safety and risk assessment. This section aims to demystify the lexicon of radiation measurement, providing a clear understanding of the units used and their significance.
Counts Per Minute (CPM): A Basic Indicator
Counts Per Minute (CPM) serves as a fundamental, easily accessible metric in radiation detection. It represents the number of ionizing events detected by a radiation instrument in a one-minute interval.
While CPM provides a preliminary indication of radiation activity, it's crucial to recognize its limitations. CPM values are highly dependent on the type of detector used and the specific radiation being measured, therefore it is a relative measurement.
Radioactivity: The Source of Emission
Radioactivity describes the phenomenon where unstable atomic nuclei spontaneously decay, releasing energy and particles in the form of radiation. This decay process transforms the original atom into a different atom, often of a different element.
The rate at which a radioactive substance decays is a key characteristic, described by its half-life. Half-life is the time it takes for half of the radioactive atoms in a sample to decay.
Different radioactive isotopes exhibit vastly different half-lives, ranging from fractions of a second to billions of years.
Key Units of Radiation Measurement
To rigorously quantify radiation, specific units have been established to characterize its different aspects. These units are standardized to ensure consistent and reliable measurements across scientific, industrial, and regulatory contexts.
Becquerel (Bq): Quantifying Radioactive Decay
The Becquerel (Bq) is the SI unit of radioactivity. One Becquerel is defined as one radioactive decay event per second.
This unit directly quantifies the rate at which a radioactive substance is disintegrating. For instance, a sample with an activity of 100 Bq undergoes 100 decay events each second.
Sievert (Sv): Assessing Health Effects
The Sievert (Sv) is the unit of equivalent dose and effective dose, which are used to quantify the potential health effects of radiation exposure. It takes into account not only the amount of energy deposited in tissue but also the type of radiation and the sensitivity of different organs to radiation.
Therefore, the Sv is a crucial unit for assessing the risk of radiation-induced health problems, like cancer.
Millisievert (mSv) and Microsievert (µSv): Practical Dose Assessment
The Millisievert (mSv) and Microsievert (µSv), representing one-thousandth and one-millionth of a Sievert respectively, are commonly used in practical radiation protection. These units provide a more manageable scale for expressing typical radiation doses encountered in everyday life and occupational settings.
For example, the dose from a typical chest X-ray is on the order of mSv, while background radiation levels are often measured in µSv per hour.
Gray (Gy): Measuring Absorbed Dose
The Gray (Gy) is the SI unit of absorbed dose, representing the amount of energy deposited by ionizing radiation per unit mass of a substance.
It is a physical quantity that describes the energy transfer from radiation to matter. Unlike the Sievert, the Gray does not account for the type of radiation or the sensitivity of different tissues.
The distinction between Gray and Sievert is essential. The Gray measures the physical energy deposition, while the Sievert adjusts this value to reflect the biological impact of that energy. This adjustment depends on the type of radiation, because some types of radiation are more damaging per unit of energy deposited.
Radiation Exposure and Dose: Understanding the Impact
Building upon the fundamental understanding of radiation, we now turn to a critical distinction: the difference between exposure and dose. These terms, while often used interchangeably, represent fundamentally different aspects of how radiation interacts with matter, particularly living organisms. Grasping these nuances is essential for accurately assessing potential risks and implementing effective safety measures.
Defining Radiation Dose
Dose refers to the quantity of radiation energy absorbed by a specific mass of material, such as human tissue.
It is a direct measure of the energy deposited within the body, and it's this energy deposition that leads to potential biological effects. The Gray (Gy) is the SI unit for absorbed dose, representing one joule of energy absorbed per kilogram of mass.
Clarifying Radiation Exposure
Exposure, on the other hand, describes the amount of ionization produced in air by ionizing radiation. It is a measure of the radiation field itself, quantifying the amount of ionization radiation creates in a given volume of air.
Exposure is typically measured in Coulombs per kilogram (C/kg) in the SI system, and it provides an indication of the radiation's intensity.
It’s important to note that exposure does not directly quantify the energy absorbed by a person or object; rather, it describes the radiation field to which they are subjected.
The Nuance of Effective Dose
While absorbed dose measures the energy deposited in specific tissues, the concept of effective dose provides a more comprehensive assessment of radiation risk. Effective dose attempts to account for the varying sensitivities of different organs and tissues to radiation.
Certain organs, such as the bone marrow and thyroid, are more susceptible to radiation damage than others. Effective dose is calculated by weighting the absorbed dose in each organ or tissue by a tissue weighting factor (Wt), which reflects its relative radiosensitivity.
The Sievert (Sv) is the unit used for effective dose. This allows for the calculation of a whole-body dose that considers the varied risk posed by radiation to different parts of the body.
Significance of Weighing Factors
The use of weighting factors in calculating effective dose is crucial for several reasons:
-
Risk Assessment: It allows for a more accurate estimation of the overall risk of radiation-induced health effects, particularly cancer.
-
Regulatory Compliance: Regulatory bodies use effective dose as a key metric for setting radiation safety standards and limits.
-
Optimization of Protection: It enables the optimization of radiation protection measures by focusing on the tissues and organs most at risk.
Dose vs. Exposure: Why the Distinction Matters
Understanding the difference between dose and exposure is vital for interpreting radiation measurements and assessing potential health risks:
-
Exposure measures the strength of the radiation field; dose measures the energy deposited within a material.
-
Exposure is useful for characterizing radiation sources; dose is more directly related to biological effects.
-
While exposure can be used to estimate dose, it is not a direct substitute.
Application in Practice
In practical radiation protection, both exposure and dose measurements are essential. Exposure measurements are used to assess the intensity of radiation fields and to ensure that radiation sources are properly controlled. Dose measurements, or estimations thereof, are used to assess the potential health risks to individuals and to ensure that radiation exposure limits are not exceeded.
In conclusion, a comprehensive understanding of radiation exposure and dose, including the nuances of effective dose, is indispensable for professionals in fields such as radiation safety, medical imaging, and nuclear energy. By accurately quantifying and interpreting these measurements, we can effectively mitigate potential risks and ensure the safe use of radiation technologies.
Background Radiation: An Inevitable Presence
Having established the units and principles of radiation measurement, it's crucial to understand that we are constantly exposed to low levels of radiation from natural sources. This background radiation forms a baseline against which any additional exposure, whether from medical procedures or other sources, must be evaluated. Understanding its origins and typical levels is paramount for accurate risk assessment and informed decision-making.
Defining Background Radiation
Background radiation is defined as the ubiquitous ionizing radiation present in the environment from natural sources. It represents the radiation exposure we receive daily, regardless of our occupation or activities. This constant exposure stems from sources both terrestrial and extraterrestrial, shaping the very fabric of our radiological environment.
Sources of Background Radiation
The sources of background radiation are diverse, reflecting the complex interplay of geological and cosmic processes. These sources can be broadly categorized, each contributing a varying degree to our overall exposure.
Cosmic Rays: Radiation from Space
Cosmic rays, high-energy particles originating from outside the Earth's atmosphere, continuously bombard our planet. These particles interact with the atmosphere, producing a cascade of secondary particles that contribute to the overall radiation dose at ground level. The intensity of cosmic radiation varies with altitude and latitude, being more intense at higher altitudes and closer to the poles due to the Earth's magnetic field. Therefore, air travel increases a person's annual exposure.
Terrestrial Radiation: Naturally Occurring Radioactive Materials (NORM)
The Earth's crust contains naturally occurring radioactive materials (NORM), such as uranium, thorium, and potassium-40. These radioactive elements are present in varying concentrations in soil, rocks, and water, leading to external exposure through direct radiation and internal exposure through ingestion and inhalation.
Specific geological formations, such as granite-rich areas, tend to exhibit higher levels of terrestrial radiation. The building materials sourced from these areas also contribute to background exposure.
Radon Gas: An Indoor Threat
Radon is a colorless, odorless, and radioactive gas produced by the decay of uranium in soil and rocks. It seeps into buildings through cracks in foundations and other entry points. Radon is a significant contributor to background radiation exposure, particularly indoors, and is considered the second leading cause of lung cancer after smoking. Effective ventilation and radon mitigation measures are essential to minimize indoor radon levels.
Internal Exposure: Radiation Within
Radioactive materials are also present within our bodies. Potassium-40, for instance, is a naturally occurring radioactive isotope found in all living organisms, contributing to internal radiation exposure. The levels are generally low and considered a natural part of biological processes.
Typical Levels of Background Radiation
Background radiation levels vary depending on location, altitude, and geological composition. However, typical annual doses range from 1.5 to 3.5 mSv (millisieverts) worldwide. Understanding these baseline levels is crucial for contextualizing radiation measurements from other sources and accurately assessing potential risks.
Monitoring these levels serves as a crucial element for environmental safety and public health. It enables the accurate quantification of any additional radiation exposure from other sources, ensuring a comprehensive approach to radiation management and the promotion of public safety.
Measurement Tools and Techniques: Detecting and Quantifying Radiation
Having established the units and principles of radiation measurement, it’s imperative to explore the array of tools and techniques scientists and technicians employ to detect and quantify radiation. These instruments are not merely passive observers; they are active participants in ensuring safety, conducting research, and monitoring environmental conditions.
This section provides an overview of the operational mechanisms and distinct applications of these tools.
Geiger Counters: Ubiquitous Radiation Detectors
The Geiger Counter, formally known as the Geiger-Müller tube, stands as one of the most recognizable and widely used radiation detectors. Its principle of operation is elegantly simple yet remarkably effective.
The device consists of a tube filled with an inert gas, typically helium, neon, or argon, at low pressure. A high voltage is applied between the tube's anode and cathode. When ionizing radiation enters the tube, it collides with the gas atoms, knocking electrons loose.
These liberated electrons are then accelerated towards the anode, causing a cascade of further ionizations, resulting in an electrical pulse.
This pulse is registered as a count, and the counts per minute (CPM) are displayed, providing a direct indication of the radiation level. Geiger counters are particularly effective at detecting beta and gamma radiation.
Their robustness and relative simplicity make them invaluable tools for initial radiation surveys and contamination monitoring.
However, they possess limitations. Geiger counters typically do not differentiate between types or energies of radiation, and they can become saturated at high radiation levels, leading to inaccurate readings.
Scintillation Detectors: High-Precision Measurements
Scintillation detectors represent a more sophisticated approach to radiation measurement, offering enhanced sensitivity and the ability to distinguish between different types of radiation.
These detectors utilize materials, such as sodium iodide or plastic scintillators, that emit light when struck by ionizing radiation.
The emitted light, proportional to the energy of the incident radiation, is then detected by a photomultiplier tube (PMT).
The PMT converts the light into an electrical signal, which is amplified and analyzed.
The amplitude of the signal is directly related to the energy of the radiation, allowing for spectroscopic analysis.
Scintillation detectors are employed in a diverse range of applications, including medical imaging (e.g., PET scans), homeland security (e.g., portal monitors), and scientific research.
Their ability to provide detailed energy information makes them essential for identifying specific radioactive isotopes and characterizing radiation fields.
A Spectrum of Radiation Detectors and Monitors
Beyond Geiger counters and scintillation detectors lies a broad spectrum of specialized radiation detectors, each tailored to specific applications and radiation types.
-
Ionization Chambers offer precise measurements of radiation dose and are frequently used in radiation therapy and calibration laboratories.
-
Proportional Counters provide energy resolution superior to Geiger counters, enabling the differentiation of alpha and beta particles.
-
Neutron Detectors are specifically designed to detect neutron radiation, crucial in nuclear reactor monitoring and safeguards.
The selection of an appropriate detector depends heavily on the specific measurement requirements, including the type of radiation, the energy range of interest, and the desired level of accuracy.
Survey Meters: On-Site Radiation Assessment
Survey meters are portable instruments designed for rapid assessment of radiation levels in the field. These devices, often incorporating Geiger-Müller tubes or scintillation detectors, are used to scan areas for contamination, identify radiation sources, and ensure compliance with safety regulations.
Survey meters are indispensable tools in nuclear facilities, environmental monitoring sites, and emergency response situations.
They allow personnel to quickly identify areas of elevated radiation and take appropriate protective measures.
Regular calibration and maintenance are crucial to ensure the accuracy and reliability of survey meters.
Dosimeters: Personal Radiation Monitoring
Dosimeters are small, wearable devices used to measure an individual's cumulative radiation exposure over a period of time.
These devices are essential for workers in radiation-related industries, providing a record of their exposure to ensure compliance with regulatory limits.
Two common types of dosimeters are film badges and thermoluminescent dosimeters (TLDs). Film badges utilize radiation-sensitive film that darkens upon exposure, while TLDs store energy when exposed to radiation and release it as light upon heating.
The amount of light emitted is proportional to the radiation dose.
Electronic personal dosimeters (EPDs) offer real-time dose monitoring and alarm capabilities, providing immediate feedback to the wearer.
Principles of Radiation Safety: Minimizing Risk
Having established the units and principles of radiation measurement, it’s imperative to explore the array of tools and techniques scientists and technicians employ to detect and quantify radiation. These instruments are not merely passive observers; they are active participants in a continuous effort to safeguard human health and the environment from the potential hazards of ionizing radiation. However, the most advanced detection technology is ultimately subservient to the core philosophies that guide its prudent deployment. At the heart of these philosophies lies the principle of minimizing risk.
The ALARA Imperative: A Cornerstone of Radiation Protection
The ALARA principle, an acronym for "As Low As Reasonably Achievable," is a fundamental tenet in radiation safety. It's more than just a regulatory requirement; it's an ethical obligation to minimize radiation exposure, taking into account social, economic, and technical factors. It dictates that even if exposure levels are below regulatory limits, efforts should still be made to reduce them further, if reasonably possible.
Defining "Reasonably Achievable"
The crucial element of ALARA is the concept of "reasonably achievable." This isn't simply a matter of minimizing cost. It necessitates a comprehensive cost-benefit analysis that weighs the reduction in radiation dose against the expense and effort required to achieve it.
This analysis must consider a multitude of factors, including the available technology, the economic implications, the social impact, and the specific context of the radiation source and potential exposure pathways.
The Three Pillars of ALARA: Time, Distance, Shielding
The practical application of ALARA rests on three foundational pillars: minimizing time, maximizing distance, and utilizing shielding. These strategies are not mutually exclusive; rather, they are often employed in concert to achieve the most effective dose reduction.
-
Time: Reducing the duration of exposure directly reduces the total dose received. This can involve optimizing work procedures, utilizing remote handling equipment, or scheduling activities in areas with lower radiation fields.
-
Distance: Radiation intensity decreases dramatically with distance from the source. This is governed by the inverse square law. Therefore, maintaining a safe distance is paramount. Even a small increase in distance can lead to a significant reduction in dose.
-
Shielding: Inserting shielding materials between the radiation source and the individual can effectively attenuate radiation. Common shielding materials include lead, concrete, and water, chosen based on the type and energy of radiation. The selection of shielding depends on factors like cost, weight, and practicality.
Implementing ALARA in Practice
The ALARA principle is not merely a theoretical concept; it's a practical framework that guides the design, operation, and maintenance of facilities and equipment involving radiation. Its effective implementation requires a proactive and systematic approach.
Workplace Protocols and Training
In practical settings, ALARA translates into strict workplace protocols. These include regular safety training for personnel, clear operational procedures, and the use of personal protective equipment (PPE) such as dosimeters, gloves, and respirators.
Workers must be educated about the risks associated with radiation exposure. They should know how to use equipment safely, and how to identify and report potential hazards.
Engineering Controls and Monitoring
Engineering controls, such as automated systems, remote handling devices, and robust ventilation systems, play a crucial role in minimizing exposure. Continuous monitoring of radiation levels is essential to identify potential leaks or breaches in containment.
Routine inspections and maintenance of equipment help to ensure that radiation safety systems are functioning correctly and effectively.
A Culture of Safety
Ultimately, the successful implementation of ALARA depends on fostering a strong safety culture within an organization. This culture must prioritize the well-being of workers and the public, promote open communication, and encourage continuous improvement in radiation safety practices.
It is the responsibility of everyone involved in activities involving radiation to embrace the ALARA principle. That will ensure that radiation exposure is kept "As Low As Reasonably Achievable." The pursuit of ALARA is an ongoing process, demanding diligence, vigilance, and a commitment to continuous improvement.
Health Effects of Radiation: Understanding the Risks
Having established the principles of radiation safety and the ALARA approach, it's essential to confront the tangible consequences of radiation exposure on human health. While radiation plays a vital role in medicine and industry, its potential to induce harm necessitates a thorough understanding of its effects, especially regarding cancer and the contentious realm of low-level exposure.
Cancer Risk and Ionizing Radiation
The most widely recognized health risk associated with ionizing radiation is an increased probability of developing cancer. This elevated risk isn't a certainty, but rather a statistical increase above the baseline cancer rate within a population. The mechanisms by which radiation induces cancer are complex, involving direct DNA damage and the generation of reactive oxygen species that can further disrupt cellular processes.
Mechanisms of Radiation-Induced Cancer
Ionizing radiation possesses sufficient energy to directly damage DNA molecules, potentially leading to mutations.
These mutations, if not repaired correctly, can initiate or promote the development of cancerous cells. Furthermore, radiation can induce epigenetic changes that alter gene expression, contributing to tumor formation.
Types of Cancers Linked to Radiation Exposure
While radiation exposure can potentially increase the risk of various cancers, certain types have shown a stronger association. Leukemia, particularly acute myeloid leukemia, has been linked to relatively high doses of radiation, as observed in studies of atomic bomb survivors.
Thyroid cancer is another concern, especially in children exposed to radioactive iodine. Other cancers, like breast, lung, and colon cancer, have also been associated with radiation exposure in epidemiological studies, though the relationship can be complex and influenced by other factors.
The Controversy of Low-Level Radiation Exposure
The health effects of high-dose radiation are relatively well-established, primarily through studies of atomic bomb survivors and radiation accidents. However, the effects of low-level radiation exposure (LLRE), defined as doses comparable to or slightly above background levels, remain a subject of ongoing debate and scientific scrutiny.
The Linear No-Threshold (LNT) Model
The prevailing model used to estimate cancer risk from LLRE is the Linear No-Threshold (LNT) model. This model posits that any amount of radiation, no matter how small, carries a proportional risk of cancer, with no threshold below which the risk is zero.
This model is often used for regulatory purposes, providing a conservative approach to radiation protection.
Challenges to the LNT Model
The LNT model has faced challenges from some scientists who argue that it overestimates the risk of LLRE. Some studies have suggested a hormetic effect, where low doses of radiation may actually be beneficial, stimulating cellular repair mechanisms and enhancing the immune system.
However, the evidence supporting hormesis is still limited and controversial. Other researchers propose that there may be a threshold below which radiation exposure does not significantly increase cancer risk.
Ongoing Research and Uncertainties
Research on the health effects of LLRE is ongoing, with scientists employing various approaches, including epidemiological studies, cellular and molecular biology research, and animal experiments.
These studies aim to better understand the mechanisms of radiation-induced damage at low doses and to refine risk assessment models. It's crucial to acknowledge the uncertainties that still exist in this field and to remain open to new evidence as it emerges.
The Precautionary Principle and Risk Management
Given the uncertainties surrounding LLRE, a precautionary approach is often adopted in radiation protection. This means taking measures to minimize radiation exposure even when the risks are not fully understood, particularly for vulnerable populations like children and pregnant women.
Effective risk communication is also essential, helping the public understand the potential risks and benefits of radiation-related technologies while fostering informed decision-making.
Regulations and Guidelines: Ensuring Safety and Compliance
Having established the principles of radiation safety and the ALARA approach, it's essential to confront the tangible consequences of radiation exposure on human health. While radiation plays a vital role in medicine and industry, its potential to induce harm necessitates a thorough understanding of the regulatory landscape designed to mitigate risks and ensure the well-being of both the public and radiation workers. This section outlines the framework of regulations and guidelines established to protect individuals from excessive radiation exposure, emphasizing the critical importance of adhering to safe limits.
Maximum Permissible Dose (MPD) and Public Safety
The cornerstone of radiation protection is the concept of the Maximum Permissible Dose (MPD), a dose limit established by regulatory bodies to define safe exposure thresholds for the general population. The MPD represents the amount of radiation that an individual can receive over a specific period (typically a year) without incurring a significant risk of adverse health effects.
It's important to understand that the MPD is not a guarantee of absolute safety, but rather a level deemed acceptable based on current scientific understanding and risk assessment. Regulatory bodies such as the International Commission on Radiological Protection (ICRP) and national agencies (e.g., the U.S. Nuclear Regulatory Commission, NRC) continuously review and update these limits as new data emerges.
The establishment of MPDs reflects a balancing act between the benefits derived from radiation-related activities (e.g., medical imaging, nuclear power) and the potential risks. The goal is to allow for the responsible use of radiation while minimizing the potential for harm to the population.
Occupational Exposure Limits: Protecting Radiation Workers
Individuals working in radiation-related industries, such as nuclear power plants, hospitals (radiology departments), and research laboratories, are potentially exposed to higher levels of radiation than the general public. To protect these workers, specific Occupational Exposure Limits are established, which are typically higher than the MPD for the general public but still significantly below levels considered immediately dangerous.
These limits recognize the inherent risks associated with radiation work while allowing workers to perform their duties effectively. Occupational exposure limits are usually accompanied by comprehensive radiation safety programs, including:
- Regular radiation monitoring.
- The use of personal protective equipment (PPE).
- Training on radiation safety procedures.
The implementation and enforcement of occupational exposure limits are crucial for safeguarding the health of radiation workers and preventing long-term health consequences. Furthermore, these limits are often supplemented by internal dose limits, which account for the intake of radioactive materials into the body.
Public Exposure Limits: Minimizing Environmental Impact
Beyond individual dose limits, regulations also address the overall impact of radiation sources on the environment and the general public. Public Exposure Limits govern the release of radioactive materials into the environment from nuclear facilities, industrial operations, and other sources.
These limits are designed to ensure that the collective dose to the public remains within acceptable bounds, considering factors such as:
- Population density near radiation sources.
- The potential for environmental contamination.
- The pathways through which radiation can reach individuals (e.g., air, water, food).
Compliance with public exposure limits often requires sophisticated monitoring and control technologies, as well as rigorous environmental assessments. Effective regulation in this area is essential for maintaining public trust and ensuring the long-term sustainability of radiation-related activities. Adhering to these regulations ensures a safer environment for everyone.
Video: CPM Radiation: Safe Levels, Risks & Exposure
FAQs about CPM Radiation
What does "CPM" mean in the context of radiation?
CPM stands for "counts per minute." It's a measurement of how many radioactive decay events are detected by a radiation detector in one minute. Higher cpm radiation generally indicates a stronger radioactive source or higher levels of exposure.
What is considered a "safe" level of cpm radiation?
There's no single universally "safe" cpm radiation level. Background radiation varies significantly by location. Safe levels also depend on the specific radioactive material and exposure duration. Regulatory bodies set limits for occupational and public exposure, aiming to minimize risk.
What are the risks associated with exposure to high cpm radiation?
Exposure to high cpm radiation can damage cells and DNA. Short-term, high-level exposure may cause radiation sickness. Long-term exposure increases the risk of cancer and other health problems. The severity of these effects depends on the dose and duration of the cpm radiation exposure.
How can I minimize my exposure to cpm radiation?
Minimizing exposure involves several strategies: distance, shielding, and time. Increase your distance from the source, use appropriate shielding materials, and limit the time spent near radiation. Following safety protocols in radiation-related occupations is crucial for controlling cpm radiation exposure.
So, there you have it! CPM radiation might sound scary, but understanding the safe levels and potential risks can help you make informed decisions and stay aware of your environment. Remember, a little knowledge goes a long way in navigating the world around us, so stay curious and keep learning about how cpm radiation impacts our daily lives!