Scientific Definition of Property: Decoding It
In the realm of legal theory, the scientific definition of property represents more than a simple ownership claim; it involves a complex interplay of rights, responsibilities, and economic considerations. The Coase Theorem, a cornerstone in understanding property rights, posits that clearly defined property rights can lead to efficient resource allocation, regardless of the initial distribution. Organizations like the World Intellectual Property Organization (WIPO) actively work to standardize and protect intellectual property rights globally, highlighting the importance of formalized definitions. Hernando de Soto, through his work on property rights in developing nations, underscores how the lack of clear legal definitions can hinder economic progress, emphasizing the need for a scientific approach. These concepts contrast with commons-based resources, which do not have exclusive private ownership, illustrating the critical role of scientific definitions in maintaining economic stability and encouraging innovation.
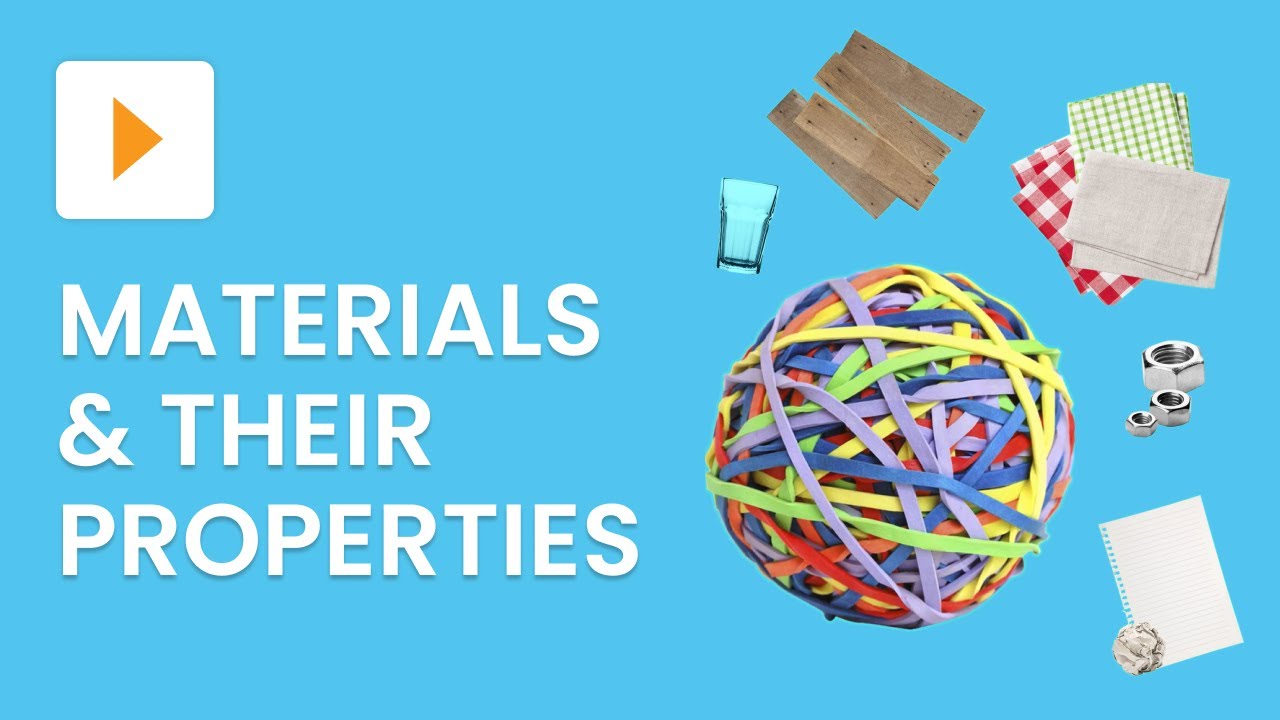
Image taken from the YouTube channel ClickView , from the video titled Materials And Their Properties .
Matter and measurement are inextricably linked, forming the bedrock of scientific inquiry and technological advancement. Our ability to understand the universe around us, from the smallest subatomic particle to the largest celestial body, hinges on our capacity to both define what matter is and how to quantify its properties.
The Symbiotic Dance of Matter and Measurement
The relationship between matter and measurement is not a one-way street. It is a dynamic and iterative process where each informs and refines the other. The very definition of matter – anything that has mass and occupies space – implicitly necessitates measurement. Mass, a fundamental property of matter, requires precise quantification. Similarly, the space that matter occupies – its volume – can only be determined through measurement techniques.
Conversely, our understanding of matter dictates the development and refinement of measurement tools and methodologies. As our knowledge of matter expands, we are driven to create increasingly sophisticated instruments capable of probing its intricacies with greater accuracy and precision.
Navigating the Landscape of Scientific Inquiry
This exploration into the scientific definition of property will delve into the core disciplines that underpin our understanding of matter. We will examine the contributions of physics, chemistry, materials science, metrology, and statistics. Each discipline offers a unique perspective and set of tools for characterizing matter.
We will navigate the fundamental laws governing matter and energy, the composition and structure of molecules, the relationship between material structure and properties, the science of measurement, and the statistical analysis of experimental data. This holistic approach aims to provide a comprehensive understanding of how scientists define and quantify the properties of matter.
The Enduring Significance Across Disciplines
The insights gained from this exploration are not confined to the realm of pure science. A robust understanding of matter and its properties is crucial in engineering, technology, and numerous other fields.
Engineers rely on material property data to design structures, devices, and systems. Technological advancements depend on the ability to manipulate and control the properties of matter. From developing new materials for aerospace applications to creating more efficient energy storage solutions, a deep understanding of matter is essential for innovation and progress.
Foundational Disciplines: A Multi-Faceted Approach to Understanding Matter
Our comprehension of matter is not born from a single field, but rather through the convergence of several scientific disciplines. Each discipline offers a unique lens through which we can analyze and define the properties of matter. Physics lays the groundwork by elucidating the fundamental laws governing matter and energy, while chemistry delves into the molecular composition and reactions that dictate material behavior. Materials science bridges the gap by exploring the intricate relationship between a material's structure and its resulting properties. All the while, metrology provides the rigorous framework for precise measurement, and statistics enables us to extract meaningful insights from experimental data.
The Interwoven Tapestry of Scientific Disciplines
These disciplines are not isolated entities; they are deeply interconnected, forming a rich and complex tapestry of scientific understanding. Progress in one area often spurs advancements in others, creating a synergistic relationship that drives innovation. For example, breakthroughs in quantum mechanics (physics) have revolutionized our understanding of chemical bonding (chemistry), which in turn has led to the design of novel materials with tailored properties (materials science). The accurate measurement of these properties (metrology) and the statistical analysis of experimental results are crucial for validating theoretical models and guiding further research.
Physics: Unveiling the Fundamental Laws
Physics provides the bedrock upon which our understanding of matter is built. It seeks to identify the fundamental laws that govern the behavior of matter and energy at all scales.
Classical Mechanics: Matter in Motion
Classical mechanics, pioneered by Isaac Newton, describes the motion of macroscopic objects under the influence of forces. It provides the foundation for understanding concepts such as inertia, momentum, and energy conservation.
Electromagnetism: The Dance of Charges
Electromagnetism, unified by James Clerk Maxwell, explores the interaction between electric and magnetic fields. It explains phenomena such as light, radio waves, and the behavior of charged particles in materials.
Thermodynamics: Energy in Transition
Thermodynamics deals with the relationships between heat, work, and energy. It provides the framework for understanding energy transfer and the behavior of systems at equilibrium, concepts vital to materials processing and chemical reactions.
Quantum Mechanics: Matter at the Atomic Level
Quantum mechanics delves into the realm of atoms and subatomic particles. It explains the wave-particle duality of matter, the quantization of energy, and the probabilistic nature of physical phenomena. This is crucial for understanding chemical bonding and the electronic properties of materials.
Chemistry: Exploring Molecular Structure and Reactions
Chemistry focuses on the composition, structure, properties, and reactions of matter at the atomic and molecular level. It provides the tools and knowledge to manipulate matter and create new substances with desired properties.
Understanding chemical bonding, molecular structure, and reaction mechanisms is essential for designing new materials and optimizing chemical processes. Chemistry provides the language and concepts to describe how atoms combine to form molecules and how these molecules interact with each other.
Materials Science: Engineering Matter for Specific Purposes
Materials science bridges the gap between fundamental science and engineering applications. It focuses on the relationship between the structure and properties of materials. This allows for the design and development of new materials with tailored properties for specific applications.
Materials scientists investigate how the arrangement of atoms and molecules within a material affects its mechanical, thermal, electrical, and optical properties. They also study how processing techniques can be used to manipulate the structure of materials and optimize their performance.
Metrology: The Science of Accurate Measurement
Metrology is the science of measurement. It ensures the accuracy, reliability, and traceability of measurements used to characterize matter. Metrology plays a crucial role in validating scientific theories, ensuring product quality, and facilitating international trade.
Metrologists develop and maintain measurement standards, calibrate instruments, and develop new measurement techniques. Their work is essential for ensuring that measurements are consistent and comparable across different laboratories and industries.
Statistics: Extracting Meaning from Data
Statistics provides the tools and methods for collecting, analyzing, interpreting, and presenting data. It is essential for quantifying uncertainties in measurements, validating experimental results, and making informed decisions based on data.
Statistical analysis allows scientists and engineers to identify patterns in data, test hypotheses, and estimate the reliability of their findings. It is a crucial tool for understanding the inherent variability of matter and the limitations of measurement techniques.
Pioneering Minds: Key Figures in Unraveling the Mysteries of Matter
The understanding we possess today about matter is not a spontaneous creation, but the cumulative result of centuries of dedicated inquiry by brilliant scientists. These pioneering individuals, through their groundbreaking theories and meticulous experimentation, have reshaped our perception of the physical world. Their insights have not only expanded the horizons of scientific knowledge but have also laid the foundation for technological advancements that define modern civilization.
The Architects of Our Understanding
This section pays homage to some of the most influential figures whose contributions have been instrumental in deciphering the mysteries of matter. We'll explore their key discoveries and examine how their work has indelibly shaped our contemporary scientific understanding.
Isaac Newton: The Foundation of Classical Physics
Isaac Newton's laws of motion and universal gravitation stand as a cornerstone of classical physics. His Principia Mathematica revolutionized the way we understand the movement of objects. Newton's work provided a comprehensive framework for explaining phenomena ranging from the trajectory of a projectile to the orbits of planets.
Newton's laws provided the foundation for mechanics and engineering and paved the way for subsequent advancements in physics and technology.
James Clerk Maxwell: Unifying Electricity and Magnetism
James Clerk Maxwell achieved a monumental feat by unifying electricity and magnetism into a single electromagnetic force. His Maxwell's equations not only explained the behavior of electric and magnetic fields but also predicted the existence of electromagnetic waves. This prediction laid the groundwork for technologies such as radio, television, and wireless communication.
Maxwell's insights into the nature of light as an electromagnetic wave revolutionized optics and spectroscopy, forever changing how we understand matter's interaction with electromagnetic radiation.
Josiah Willard Gibbs: The Architect of Chemical Thermodynamics
Josiah Willard Gibbs is considered one of the most important scientists in American history and the father of chemical thermodynamics. His work provided a rigorous mathematical framework for understanding chemical equilibrium, phase transitions, and the relationship between energy, entropy, and chemical potential. Gibbs' phase rule is an invaluable tool for predicting the behavior of multi-component systems and understanding the conditions under which different phases can coexist in equilibrium.
His work remains central to materials science, chemical engineering, and many other fields.
Albert Einstein: Revolutionizing Space, Time, and Matter
Albert Einstein's theory of relativity irrevocably altered our understanding of space, time, gravity, and matter. His famous equation, E=mc², revealed the equivalence of mass and energy, demonstrating that matter can be converted into energy and vice versa. General relativity redefined gravity not as a force but as a curvature of spacetime caused by mass and energy.
Einstein's work had a profound impact on cosmology, nuclear physics, and particle physics, transforming our understanding of the universe at its most fundamental level.
The Quantum Revolutionaries: Planck, Schrödinger, Heisenberg
The advent of quantum mechanics in the early 20th century ushered in a new era in our understanding of matter at the atomic and subatomic levels. Max Planck's hypothesis of quantized energy marked the birth of quantum theory.
Erwin Schrödinger formulated the Schrödinger equation, a fundamental equation in quantum mechanics that describes the time evolution of quantum systems. Werner Heisenberg's uncertainty principle demonstrated the inherent limits to the precision with which certain pairs of physical properties, such as position and momentum, can be simultaneously known.
These three figures established the quantum mechanical description of matter.
Linus Pauling: Unveiling the Secrets of Chemical Bonding
Linus Pauling's work on chemical bonding and molecular structure revolutionized chemistry. His concept of electronegativity provided a framework for understanding the nature of chemical bonds and predicting the properties of molecules. Pauling also made significant contributions to the understanding of protein structure, paving the way for advancements in molecular biology and medicine.
Richard Feynman: A Master of Quantum Electrodynamics
Richard Feynman was a brilliant theoretical physicist known for his contributions to quantum electrodynamics (QED). His Feynman diagrams provided a powerful tool for visualizing and calculating the interactions between elementary particles. Feynman also made significant contributions to the understanding of superfluidity and particle physics. His lectures on physics are renowned for their clarity and insight.
Properties of Matter: A Comprehensive Overview
Matter, in its diverse forms, is characterized by a multitude of properties that dictate its behavior and utility. These properties are not merely descriptive attributes; they are the fundamental determinants of how matter interacts with its environment and with other forms of matter. Understanding these properties is crucial for scientists, engineers, and technologists in designing materials, predicting their performance, and tailoring them for specific applications.
This section will provide a detailed exploration of the various properties of matter, categorized into distinct types. We will delve into the significance of each category and discuss how these properties collectively influence the behavior and applications of materials across diverse fields.
Categorizing the Intrinsic Qualities of Matter
Properties of matter can be broadly categorized into physical, chemical, mechanical, thermal, electrical, magnetic, optical, acoustic, and thermodynamic properties. Each category reflects a specific aspect of how matter manifests and responds to external conditions or interactions.
The following is a breakdown of each category, describing its significance and key examples.
Physical Properties: The Observable Characteristics
Physical properties are those that can be observed or measured without changing the chemical composition of the substance. These characteristics are often readily apparent and provide initial insights into the nature of the material.
Key examples include:
- Density: A measure of mass per unit volume, crucial for buoyancy and material selection.
- Color: The visual perception of reflected or transmitted light, used for identification and aesthetic purposes.
- Melting Point: The temperature at which a solid transforms into a liquid, vital for thermal processing and stability assessments.
- Boiling Point: The temperature at which a liquid transitions to a gas, important for distillation and chemical reactions.
- Hardness: Resistance to indentation or scratching, a key factor in durability and wear resistance.
- Texture: The surface feel and appearance, influencing tactile and visual interactions.
Chemical Properties: Unveiling Reactivity
Chemical properties describe how a substance interacts with other substances, leading to changes in its chemical composition. These properties are critical for understanding chemical reactions and material stability.
Key examples include:
- Flammability: The ability to ignite and sustain combustion, a crucial safety consideration.
- Acidity/Basicity: The capacity to donate or accept protons, influencing chemical reactions and corrosion.
- Oxidation Potential: The tendency to lose electrons, determining corrosion resistance and battery performance.
- Reactivity with Water: The propensity to react with water, affecting material stability and environmental impact.
- Corrosivity: The ability to degrade materials through chemical reactions, impacting structural integrity.
- Toxicity: The potential to harm living organisms, a primary concern in material safety.
Mechanical Properties: Responding to Force
Mechanical properties describe how a material responds to applied forces, determining its structural integrity and suitability for load-bearing applications.
Key examples include:
- Strength: The ability to withstand stress without breaking or deforming, essential for structural components.
- Elasticity: The ability to return to its original shape after deformation, important for springs and flexible materials.
- Plasticity: The ability to undergo permanent deformation without fracture, utilized in forming processes.
- Hardness: Resistance to indentation or scratching, indicating wear resistance.
- Toughness: The ability to absorb energy and resist fracture, critical for impact resistance.
- Fatigue Strength: Resistance to failure under repeated loading, crucial for long-term durability.
Thermal Properties: Behavior Under Temperature Variations
Thermal properties describe how a material behaves in response to temperature changes, influencing its performance in thermal environments.
Key examples include:
- Heat Capacity: The amount of heat required to raise the temperature of a substance, vital for heat storage and transfer.
- Thermal Conductivity: The ability to conduct heat, crucial for heat sinks and insulation materials.
- Thermal Expansion: The change in size with temperature, important for designing structures that experience temperature variations.
- Melting Point: The temperature at which a solid transforms into a liquid.
- Boiling Point: The temperature at which a liquid transitions to a gas.
Electrical Properties: Conductivity and Resistivity
Electrical properties describe how a material conducts or resists the flow of electric current, essential for electrical and electronic applications.
Key examples include:
- Conductivity: The ability to conduct electric current, crucial for wires and conductive components.
- Resistivity: The resistance to the flow of electric current, important for resistors and insulators.
- Dielectric Constant: The ability to store electrical energy, utilized in capacitors.
- Semiconductivity: Intermediate conductivity, essential for transistors and integrated circuits.
- Superconductivity: Zero resistance to electric current at low temperatures, enabling lossless energy transmission.
Magnetic Properties: Responding to Magnetic Fields
Magnetic properties describe how a material responds to magnetic fields, determining its suitability for magnetic devices and applications.
Key examples include:
- Ferromagnetism: Strong attraction to magnetic fields, utilized in magnets and magnetic storage.
- Paramagnetism: Weak attraction to magnetic fields.
- Diamagnetism: Repulsion from magnetic fields.
- Magnetic Permeability: The ability to concentrate magnetic field lines, influencing inductor and transformer performance.
- Magnetic Hysteresis: The lag in magnetization behind the applied magnetic field, important for magnetic recording materials.
Optical Properties: Interacting with Light
Optical properties describe how a material interacts with light, influencing its appearance and suitability for optical applications.
Key examples include:
- Refractive Index: The measure of how much light bends when passing through a material, crucial for lenses and optical fibers.
- Absorption: The ability to absorb light, affecting color and transparency.
- Transmission: The ability to transmit light, important for windows and transparent materials.
- Reflection: The ability to reflect light, utilized in mirrors and reflective coatings.
- Luminescence: The emission of light, used in displays and lighting.
Acoustic Properties: Transmitting Sound
Acoustic properties describe how a material transmits sound, influencing its use in acoustic devices and noise control.
Key examples include:
- Speed of Sound: The rate at which sound travels through a material, important for acoustic sensors and transducers.
- Acoustic Impedance: The resistance to sound propagation, affecting sound transmission and reflection.
- Sound Absorption Coefficient: The ability to absorb sound, crucial for noise reduction and acoustic insulation.
Thermodynamic Properties: Energy, Heat, and Work
Thermodynamic properties relate to energy, heat, and work, governing the behavior of materials in thermodynamic systems.
Key examples include:
- Enthalpy: The total heat content of a system.
- Entropy: The measure of disorder or randomness in a system.
- Gibbs Free Energy: The amount of energy available to do useful work.
- Specific Heat: The amount of heat required to raise the temperature of one unit mass of a substance by one degree.
- Thermal Expansion Coefficient: The measure of how much a material expands or contracts with changes in temperature.
By understanding and manipulating these fundamental properties, scientists and engineers can unlock new possibilities in material design, leading to advancements in various fields, including medicine, energy, and technology.
Measurement Techniques: Tools for Unveiling Material Characteristics
The characterization of matter hinges on the deployment of sophisticated measurement techniques. These tools provide quantitative data that informs our understanding of material properties, enabling advancements across diverse scientific and industrial domains. Accuracy, precision, and appropriate selection of measurement methods are paramount for obtaining reliable and meaningful results.
This section will delve into essential measurement tools and techniques utilized to characterize matter. We will examine the principles underlying each technique, its applications, and provide examples of their practical implementation across various scientific and industrial settings.
Spectroscopic Methods: Deciphering Light's Interactions with Matter
Spectroscopy encompasses a suite of techniques that analyze the interaction of electromagnetic radiation with matter. By examining the emitted, absorbed, or scattered light, valuable information regarding the composition, electronic structure, and vibrational modes of a material can be obtained.
Different spectroscopic methods are sensitive to different regions of the electromagnetic spectrum, offering versatility in material characterization.
Types of Spectroscopy and Applications
UV-Vis Spectroscopy:
**Measures the absorption and transmission of ultraviolet and visible light, providing insights into electronic transitions and concentrations of substances. Commonly used in chemical analysis and quality control.
** Infrared (IR) Spectroscopy: Analyzes the absorption of infrared radiation, revealing information about molecular vibrations and functional groups. Essential for identifying organic compounds and studying chemical bonding.
Raman Spectroscopy:
**Measures the scattering of light, providing complementary information to IR spectroscopy about molecular vibrations. Useful for analyzing a wide range of materials, including polymers, semiconductors, and biological samples.
** X-ray Spectroscopy: Examines the absorption or emission of X-rays, providing information about elemental composition and chemical states. Used in materials science, surface analysis, and environmental monitoring.
Microscopy: Visualizing the Microscopic World
Microscopy techniques provide magnified images of materials, enabling the observation of microstructural features that are invisible to the naked eye. Different types of microscopes offer varying levels of magnification and resolution, allowing the visualization of features ranging from micrometers to nanometers.
The choice of microscopy technique depends on the specific material being examined and the features of interest.
Types of Microscopy and Applications
Optical Microscopy:
**Uses visible light to illuminate and magnify samples. Suitable for examining biological tissues, geological samples, and materials with relatively large microstructural features. Features are limited by the wavelength of light.
** Electron Microscopy: Employs electron beams to image samples, offering significantly higher magnification and resolution than optical microscopy. Essential for visualizing nanoscale structures and features.
Scanning Electron Microscopy (SEM):
**Scans a focused electron beam across the surface of a sample, providing high-resolution images of surface topography.
** Transmission Electron Microscopy (TEM): Transmits an electron beam through a thin sample, providing information about the internal structure and composition.
Atomic Force Microscopy (AFM):
**Scans a sharp tip across the surface of a sample to measure surface topography at the atomic level. Used for characterizing surface roughness, imaging nanomaterials, and studying biological molecules.
Calorimetry: Quantifying Heat Transfer
**Calorimetryis the science of measuring heat transfer during physical and chemical processes.Calorimeters
**are instruments used to measure the amount of heat absorbed or released during a reaction or phase transition. This technique is vital in determining the thermodynamic properties of materials and understanding reaction kinetics.
The data obtained from calorimetry can provide valuable information regarding the stability, reactivity, and energy content of materials.
Types of Calorimetry and Applications
** Differential Scanning Calorimetry (DSC): Measures the heat flow associated with phase transitions and chemical reactions as a function of temperature. Used for determining melting points, glass transition temperatures, and reaction enthalpies.
Isothermal Titration Calorimetry (ITC):
**Measures the heat released or absorbed during a titration experiment. Used for determining binding affinities and stoichiometry of molecular interactions.
Tensile Testing: Assessing Mechanical Strength
**Tensile testing machines
**are used to determine the mechanical properties of materials under tension. By applying a controlled tensile force to a sample and measuring its elongation, parameters such as strength, elasticity, and ductility can be determined. These tests are critical for ensuring the structural integrity and safety of materials used in engineering applications.
The data obtained from tensile testing are essential for material selection and design optimization.
Parameters Measured in Tensile Testing
** Tensile Strength: The maximum stress a material can withstand before breaking.
Yield Strength:
**The stress at which a material begins to deform permanently.
** Elastic Modulus: A measure of a material's stiffness.
Elongation:
**The amount a material stretches before breaking.
X-Ray Diffraction (XRD): Unveiling Crystal Structures
**X-ray diffraction (XRD)
**is a powerful technique used to determine the crystal structure and composition of materials. By analyzing the diffraction pattern of X-rays interacting with a crystalline sample, the arrangement of atoms within the crystal lattice can be determined.
XRD is widely used in materials science, chemistry, and geology for identifying crystalline phases, determining crystallite size, and assessing crystal quality.
Chromatography: Separating Mixtures into Components
**Chromatography
**is a separation technique used to separate and analyze the components of a mixture. The mixture is passed through a stationary phase, and the different components are separated based on their affinity for the stationary phase and a mobile phase.
Chromatography is an essential tool in analytical chemistry, biochemistry, and environmental science for identifying and quantifying various substances.
Types of Chromatography
** Gas Chromatography (GC): Separates volatile compounds based on their boiling points.
Liquid Chromatography (LC):
**Separates non-volatile compounds based on their polarity or size.
** Thin-Layer Chromatography (TLC): A simple and versatile technique for separating small amounts of substances.
Mass Spectrometry: Identifying Molecules by Mass
Mass spectrometry is an analytical technique used to determine the mass-to-charge ratio of ions. The sample is ionized, and the ions are separated based on their mass-to-charge ratio. This provides information about the molecular weight and structure of the components in the sample. Mass spectrometry is vital in identifying unknown compounds, determining the composition of mixtures, and studying the structure and dynamics of molecules.
Viscometry: Measuring Fluid Resistance
Viscometers are instruments used to measure the viscosity of fluids. Viscosity is a measure of a fluid's resistance to flow. Viscosity measurements are essential in various industries, including the food, petroleum, and pharmaceutical industries, for characterizing the flow properties of liquids and ensuring product quality.
Multimeters: Measuring Electrical Properties
Multimeters are versatile instruments used to measure electrical properties such as voltage, current, and resistance. They are essential tools for electrical engineers, technicians, and hobbyists for troubleshooting circuits, testing components, and measuring electrical parameters.
Profilometry: Characterizing Surface Topography
Profilometers are instruments used to measure surface roughness and topography. They scan a stylus or laser across the surface of a sample to measure the vertical deviations. Profilometry is used in manufacturing, materials science, and microelectronics for characterizing surface finish, measuring film thickness, and assessing the quality of surface treatments.
By employing these diverse measurement techniques, scientists and engineers can comprehensively characterize matter, enabling innovation and advancement across a wide spectrum of fields.
Standards and Standardization: Ensuring Accuracy and Reliability
In the realm of scientific measurement and material characterization, the importance of standards and standardization cannot be overstated. These frameworks provide the bedrock upon which accurate, reliable, and comparable data are built. Without them, the edifice of scientific progress would crumble, leading to inconsistent results, flawed conclusions, and ultimately, a breakdown in the trust that underpins the scientific method.
Standardization ensures that measurements are consistent across different laboratories, instruments, and operators, fostering collaboration and facilitating the sharing of knowledge. This section explores the crucial role of standards, the organizations that champion them, and their profound impact on science, industry, and global trade.
The Bedrock of Reliable Measurement
Standards serve as the reference points against which all measurements are compared. They define the units of measurement, ensuring that a meter in one laboratory is the same as a meter in another.
In material characterization, standards are essential for validating the performance of instruments, calibrating sensors, and ensuring the accuracy of results.
Without these benchmarks, comparing data across different sources would be akin to comparing apples and oranges, rendering meaningful analysis impossible.
Key Standardization Organizations
Several organizations worldwide are dedicated to developing and maintaining measurement standards, each playing a crucial role in ensuring consistency and comparability.
These organizations provide the infrastructure and expertise necessary to establish, disseminate, and enforce standardized practices.
National Institute of Standards and Technology (NIST) (USA)
NIST is a non-regulatory agency of the U.S. Department of Commerce. It plays a pivotal role in developing and promoting measurement, standards, and technology.
NIST's work spans a wide range of scientific and technological disciplines, from fundamental constants to advanced manufacturing.
It provides traceable measurements, calibrations, and standards to U.S. industry and scientific community.
International Bureau of Weights and Measures (BIPM)
The BIPM, based in France, is an intergovernmental organization that maintains the International System of Units (SI). The SI is the foundation for global measurement consistency.
The BIPM ensures that the SI units are accurately realized and disseminated worldwide.
It coordinates international comparisons of national measurement standards to ensure global uniformity.
International Union of Pure and Applied Chemistry (IUPAC)
IUPAC is an international organization dedicated to standardizing chemical nomenclature, terminology, and measurements.
Its work is crucial for ensuring clear communication and accurate representation of chemical information across the globe.
IUPAC recommendations are widely adopted in chemistry research, education, and industry.
International Organization for Standardization (ISO)
ISO is an independent, non-governmental international organization that develops standards for a wide range of industries and technologies. ISO standards cover everything from quality management to environmental management, and from product safety to information security.
ISO standards promote efficiency, safety, and interoperability across various sectors.
They are widely used in international trade and help to facilitate global commerce.
Universities and Research Institutions
Universities and research institutions also contribute significantly to developing new measurement techniques and standards through fundamental research.
These institutions are at the forefront of scientific innovation, pushing the boundaries of measurement science and technology.
Their research often leads to new standards and improved measurement capabilities.
The Broader Impact of Standardization
Standardized measurements have a far-reaching impact on scientific research, industrial production, and international trade.
In scientific research, standardization ensures that experimental results are reproducible and comparable, accelerating the pace of discovery.
In industrial production, standardized measurements improve product quality, reduce manufacturing costs, and enhance efficiency.
In international trade, standardized measurements facilitate the exchange of goods and services, reducing barriers and promoting economic growth.
The collective impact of these standardization efforts is a stronger and more reliable foundation for scientific progress, technological innovation, and global prosperity.
Digital Resources: Navigating the Landscape of Material Property Data
The digital age has revolutionized how we access, analyze, and utilize information across all scientific disciplines, and materials science is no exception. Vast repositories of material property data, coupled with powerful software tools, have emerged as indispensable assets for researchers, engineers, and educators alike. These resources not only streamline the process of material selection and design but also empower the development of novel materials with tailored characteristics.
This section explores the crucial role of digital resources in modern materials science, highlighting key databases, computational tools, and data analysis software that are driving innovation and accelerating scientific discovery.
The Rise of Material Property Databases
Traditional materials science relied heavily on experimental characterization and published literature to gather information on material properties. This process could be time-consuming, expensive, and often limited by the availability of data.
Today, a wealth of digital databases offers instant access to a vast collection of material properties, ranging from fundamental physical constants to complex thermodynamic behaviors. These databases are often curated by experts and regularly updated with new findings, ensuring accuracy and reliability.
Key Material Property Databases
-
Materials Project: A standout example is the Materials Project, a pioneering effort that leverages first-principles calculations based on density functional theory (DFT) to predict and store material properties. This database contains information on thousands of materials, including their crystal structures, electronic band structures, and mechanical properties. Researchers can use the Materials Project to screen potential candidates for specific applications, reducing the need for costly and time-consuming experiments.
-
PubChem (NIH): While not exclusively focused on materials, PubChem, maintained by the National Institutes of Health (NIH), serves as a comprehensive repository of chemical molecules and their associated properties. This database is invaluable for researchers working with chemical compounds and their applications in materials science. It contains a wealth of information on chemical structures, identifiers, chemical and physical properties, biological activities, patents, health, safety, toxicity data, and more.
-
ThermoML Archive: The ThermoML Archive is a specialized database focused on thermophysical property data. It provides access to experimental measurements of properties such as heat capacity, thermal conductivity, and phase equilibrium data. This archive is essential for engineers and scientists involved in process design, heat transfer calculations, and thermodynamic modeling.
Computational Tools: Predicting Material Behavior
In addition to databases, computational tools play a vital role in materials science by enabling the prediction of material properties and behaviors. These tools range from commercial software packages to open-source codes, each offering a unique set of capabilities.
-
DFT Codes: Density Functional Theory (DFT) codes are at the forefront of computational materials science. Software packages like VASP, Quantum ESPRESSO, and WIEN2k allow researchers to simulate the electronic structure of materials and predict their properties with remarkable accuracy. These codes are used to investigate a wide range of phenomena, from chemical bonding to electronic conductivity.
-
Finite Element Analysis (FEA): Finite Element Analysis is a robust computational technique used for simulating the behaviour of materials under stress, heat, or other physical conditions. FEA software, such as ANSYS or Abaqus, is widely used in engineering to optimize designs and predict the performance of materials in real-world applications.
Data Analysis Software: Extracting Insights from Experiments
The analysis of experimental data is a crucial step in materials science research. A variety of software tools are available to help researchers process, visualize, and interpret their findings.
-
Origin and MATLAB: Software packages like Origin and MATLAB provide comprehensive data analysis capabilities, including curve fitting, statistical analysis, and advanced plotting.
-
Python: Python, with its rich ecosystem of scientific libraries (such as NumPy, SciPy, and Matplotlib), has become increasingly popular for data analysis in materials science. Its open-source nature and flexibility make it an attractive choice for researchers seeking customized solutions.
The Future of Digital Resources in Materials Science
The field of digital resources for material properties is constantly evolving. New databases, computational tools, and data analysis techniques are emerging at a rapid pace. As these resources continue to improve, they will play an increasingly important role in accelerating materials discovery, optimizing material design, and advancing scientific knowledge.
Video: Scientific Definition of Property: Decoding It
FAQs: Scientific Definition of Property: Decoding It
What is a property in the scientific sense?
A property, in its scientific definition of property, is a characteristic or attribute of a substance that can be observed and measured. These observable traits help distinguish one substance from another.
How does the scientific definition of property differ from everyday use?
While we commonly use "property" to mean ownership, the scientific definition of property refers to inherent characteristics. These characteristics include things like melting point, density, or electrical conductivity.
Why is understanding the scientific definition of property important?
Understanding the scientific definition of property is crucial because it forms the basis for identifying, classifying, and understanding materials. It allows scientists to predict how materials will behave under different conditions.
What are some examples of intensive and extensive properties according to the scientific definition of property?
An intensive property, such as density or temperature, doesn't change with the amount of substance. An extensive property, like mass or volume, depends directly on the quantity of the substance present. These are both essential elements of the scientific definition of property.
So, there you have it! We've unpacked the sometimes-dense world of the scientific definition of property. Hopefully, you now have a better grasp on what scientists mean when they talk about the characteristics and attributes that make matter, well, matter. Now go forth and observe the properties all around you – science is everywhere!