Replication Fork Diagram: Guide for Students
The double helix structure of DNA, as elucidated by Watson and Crick, serves as the template for DNA replication, a process visually represented by the replication fork diagram. These diagrams, essential tools in molecular biology education, illustrate the complex interactions occurring at the replication fork, a site where DNA unwinds and new strands are synthesized. Enzymes such as DNA polymerase are critical components, catalyzing the addition of nucleotides to the growing DNA strand as depicted in the replication fork diagram. Researchers at institutions like Cold Spring Harbor Laboratory continue to refine our understanding of DNA replication, further enhancing the accuracy and utility of the replication fork diagram as an educational resource.
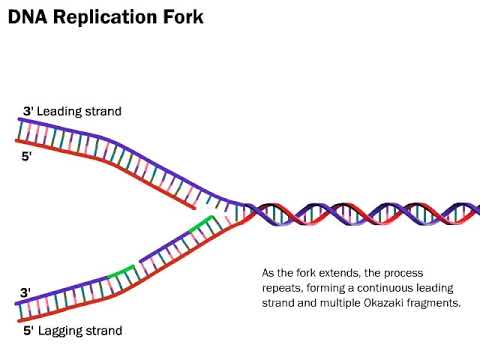
Image taken from the YouTube channel LabXchange , from the video titled DNA Replication: The Process Simplified .
DNA Replication: The Foundation of Life's Continuity
At the heart of cellular life lies the indispensable process of DNA replication. This fundamental mechanism ensures the faithful duplication of the genetic code, enabling cell division and guaranteeing the inheritance of traits across generations. Understanding DNA replication is paramount to grasping the intricacies of biology and the very essence of life itself.
The Vital Role of DNA Replication
DNA replication is not merely a biological process; it is the cornerstone of life's continuity. Without it, cell division would be impossible, rendering growth, repair, and reproduction unattainable.
Consider the implications: from a single fertilized egg developing into a complex organism, to the healing of a wound, DNA replication underpins these essential biological functions.
The process allows cells to accurately pass on their genetic material to daughter cells, ensuring genetic stability and continuity across generations. This faithful transmission of information is critical for maintaining the integrity of species and the health of individual organisms.
Semi-Conservative Replication: A Masterful Strategy
The elegance of DNA replication is further highlighted by its semi-conservative nature. This term describes how each new DNA molecule comprises one original (template) strand and one newly synthesized strand.
This mechanism, elegantly demonstrated by the Meselson-Stahl experiment, has profound implications.
The semi-conservative model offers a level of error checking and redundancy: The original strand serves as a template, guiding the synthesis of the new strand. This greatly reduces the risk of mutations accumulating over successive generations.
The original DNA is thus conserved within each daughter cell, reducing the potential for devastating genetic errors. This semi-conservative approach is a testament to the precision and efficiency of nature's designs.
DNA Replication: A Bird's Eye View of the Process
DNA replication is a complex yet remarkably orchestrated event. The entire process can be summarized in a series of key steps:
-
Initiation: Replication begins at specific sites on the DNA molecule called origins of replication.
-
Unwinding: The double helix unwinds, forming a replication fork.
-
Priming: Short RNA primers are synthesized to provide a starting point for DNA synthesis.
-
Elongation: DNA polymerase enzymes add nucleotides to the growing DNA strand, following the base-pairing rules (A with T, and C with G).
-
Termination: The process continues until the entire DNA molecule is replicated, with mechanisms to ensure accuracy and completeness.
This overview provides a roadmap for understanding the more detailed steps that follow. Each stage involves a symphony of enzymes and proteins, working in concert to ensure the accurate duplication of the genome. It is a process that highlights the beautiful complexity and exquisite precision of molecular biology.
Key Players: Enzymes and Proteins Orchestrating DNA Replication
Having established the foundational importance of DNA replication, let's turn our attention to the intricate molecular machinery that makes this process possible. A cohort of enzymes and proteins collaborates with remarkable precision to ensure faithful duplication of the genome. Each player possesses a unique role, working in concert to overcome the inherent challenges of replicating the double helix.
DNA Helicase: Unwinding the Double Helix
At the forefront of the replication process is DNA helicase, an enzyme that catalyzes the unwinding of the double helix structure of DNA. This unwinding action creates a replication fork, a Y-shaped structure that serves as the active site for DNA synthesis.
Helicase disrupts the hydrogen bonds between complementary base pairs, separating the two strands and providing access for other enzymes to initiate replication. Without helicase, the DNA strands would remain intertwined, preventing replication from proceeding.
Single-Stranded Binding Proteins (SSBPs): Preventing Re-annealing
As DNA helicase unwinds the double helix, the separated single strands become vulnerable to re-annealing, or re-pairing with each other. To prevent this, single-stranded binding proteins (SSBPs) bind to the separated strands.
SSBPs are essential for maintaining the single-stranded state of DNA. This ensures that each strand can serve as a template for the synthesis of new DNA.
Topoisomerase (DNA Gyrase): Relieving Torsional Stress
The unwinding of DNA by helicase introduces torsional stress ahead of the replication fork. This stress, if not relieved, can impede the progress of replication and even damage the DNA.
Topoisomerase, also known as DNA gyrase in prokaryotes, alleviates this torsional stress by transiently breaking and rejoining DNA strands. This allows the DNA to unwind freely, preventing supercoiling and ensuring smooth replication.
Primase: Synthesizing RNA Primers
DNA polymerase, the enzyme responsible for synthesizing new DNA strands, cannot initiate synthesis de novo. It requires a pre-existing primer to which it can add nucleotides. This is where primase comes in.
Primase is an RNA polymerase that synthesizes short RNA primers complementary to the DNA template. These primers provide a 3'-OH group, the necessary starting point for DNA polymerase to begin adding nucleotides. These short RNA primers are later replaced with DNA nucleotides.
DNA Polymerase: Synthesizing New DNA Strands
DNA polymerase is the central enzyme in DNA replication, responsible for synthesizing new DNA strands complementary to the template strands. It adds nucleotides to the 3' end of the primer, elongating the new DNA strand in the 5' to 3' direction.
Role in Elongation
DNA polymerase catalyzes the formation of phosphodiester bonds between the incoming nucleotide and the existing DNA strand. This process requires a template strand to ensure the correct nucleotide is added. The enzyme moves along the template, continuously adding nucleotides and extending the new DNA strand.
3' to 5' Exonuclease Activity (Proofreading)
DNA replication must occur with high fidelity to maintain the integrity of the genetic information. DNA polymerase possesses a 3' to 5' exonuclease activity. This activity allows it to proofread the newly synthesized DNA strand and correct any errors.
If DNA polymerase detects an incorrect nucleotide, it excises the nucleotide from the 3' end of the strand. This allows it to insert the correct nucleotide before continuing synthesis.
Processivity
Processivity refers to the ability of an enzyme to catalyze consecutive reactions without detaching from its substrate. DNA polymerase exhibits high processivity. This enables it to synthesize long stretches of DNA without frequently detaching from the template.
High processivity is crucial for efficient DNA replication, reducing the time and resources required to duplicate the genome.
DNA Ligase: Joining Okazaki Fragments
On the lagging strand, DNA synthesis occurs discontinuously, resulting in the formation of short DNA fragments called Okazaki fragments. These fragments are separated by RNA primers.
DNA ligase is the enzyme that joins these Okazaki fragments together to create a continuous DNA strand. It catalyzes the formation of a phosphodiester bond between the 3'-OH end of one fragment and the 5'-phosphate end of the adjacent fragment. This creates a seamless, continuous DNA strand.
Step-by-Step: The DNA Replication Process Unveiled
Having established the foundational importance of DNA replication, let's now dissect the process itself, a meticulously orchestrated sequence of events that ensures the faithful duplication of the genome. From the initial recognition of replication origins to the completion of daughter strands, each step is characterized by remarkable precision and coordination. A comprehensive understanding of this process requires a granular examination of its constituent phases.
Origin of Replication and the Initiation Phase
The replication process is not a spontaneous event occurring randomly across the DNA molecule. Instead, it initiates at specific, predetermined sites known as origins of replication.
These origins are characterized by unique DNA sequences that serve as recognition sites for initiator proteins.
In E. coli, the origin is termed oriC, whereas eukaryotic genomes contain multiple origins of replication to facilitate the rapid duplication of their larger genomes.
The binding of initiator proteins triggers the unwinding of the DNA double helix, creating a localized region of single-stranded DNA, which then becomes the focal point for subsequent replication events.
Replication Bubble Formation and Bidirectional Synthesis
Following the initial unwinding, the DNA strands separate further, forming a structure known as the replication bubble.
This bubble serves as the active site for DNA synthesis, with replication proceeding bidirectionally from the origin.
Bidirectional replication means that two replication forks are formed at each origin, moving in opposite directions.
This is particularly advantageous, as it effectively doubles the rate at which the DNA molecule can be replicated, crucial for efficient cell division.
The replication forks are dynamic structures, constantly extending as the DNA strands unwind and new DNA is synthesized.
Leading Strand Synthesis: The Continuous Advance
One of the two newly synthesized DNA strands, termed the leading strand, is synthesized continuously in the 5' to 3' direction.
This directionality is dictated by the inherent properties of DNA polymerase, the enzyme responsible for adding nucleotides to the growing DNA strand.
DNA polymerase can only add nucleotides to the 3' hydroxyl group of the preceding nucleotide, thereby extending the strand in a 5' to 3' fashion.
The leading strand is synthesized in one continuous piece, following the replication fork as it unwinds the DNA double helix.
This seamless synthesis contributes to the overall efficiency and accuracy of DNA replication.
Lagging Strand Synthesis: The Fragmented Approach
In contrast to the leading strand, the other newly synthesized strand, termed the lagging strand, is synthesized discontinuously.
This is again due to the 5' to 3' directionality requirement of DNA polymerase.
As the replication fork moves, the lagging strand is synthesized in short fragments known as Okazaki fragments.
Each Okazaki fragment requires a separate RNA primer to initiate DNA synthesis.
These primers are later removed and replaced with DNA, and the fragments are joined together by DNA ligase, creating a continuous strand.
The discontinuous nature of lagging strand synthesis introduces a level of complexity to the replication process, requiring additional enzymatic steps to ensure the integrity of the newly synthesized DNA.
Structural Landscape: Leading Strand, Lagging Strand, and More
Having dissected the intricate enzymatic machinery and the step-by-step progression of DNA replication, it is now prudent to shift our focus to the structural nuances inherent in this fundamental process. The differing mechanisms of leading and lagging strand synthesis, the transient role of RNA primers, and the unique challenges posed by telomeres in eukaryotic chromosomes each contribute to the fascinating structural landscape of DNA replication.
Leading Strand: The Streamlined Advance
The leading strand, characterized by its continuous synthesis, represents the simpler of the two replication modes. DNA polymerase, moving in the 5' to 3' direction along the template strand, can proceed unimpeded, adding nucleotides to the growing daughter strand as the replication fork advances.
This streamlined process minimizes the potential for errors and ensures a high fidelity of replication. The leading strand's continuous nature is a testament to the elegant design of the replication machinery.
However, it's important to acknowledge that even continuous synthesis requires meticulous coordination and proofreading to maintain genomic integrity.
Lagging Strand: A Fragmented Approach
In stark contrast to the leading strand, the lagging strand is synthesized discontinuously, in short fragments known as Okazaki fragments. This is due to the antiparallel nature of DNA and the requirement for DNA polymerase to synthesize DNA in the 5' to 3' direction.
As the replication fork opens, primase synthesizes short RNA primers on the lagging strand template. DNA polymerase then extends these primers, creating Okazaki fragments.
The inherent challenge lies in the subsequent processing of these fragments. Each fragment must be joined to its neighbor, a task accomplished by DNA ligase after the RNA primers are removed and replaced with DNA.
This discontinuous synthesis introduces opportunities for errors and requires a more complex coordination of enzymatic activities. The cyclical addition and ligation of Okazaki fragments highlights the complexity of replicating both strands simultaneously.
RNA Primer: The Necessary Catalyst
RNA primers serve as the crucial initiating points for DNA synthesis on both the leading and lagging strands. DNA polymerase requires a pre-existing 3'-OH group to add nucleotides. Primase, an RNA polymerase, fulfills this role by synthesizing short RNA sequences complementary to the DNA template.
However, the presence of RNA within the newly synthesized DNA is inherently problematic. RNA primers must be removed and replaced with DNA to maintain genomic stability. This is accomplished by specialized enzymes that excise the RNA and DNA polymerases that fill the resulting gaps.
The temporary nature of RNA primers underscores the dynamic and regulated nature of DNA replication. The replacement of RNA with DNA is a vital step in ensuring the integrity of the replicated genome.
Telomeres: Protecting the Ends
The ends of linear eukaryotic chromosomes, known as telomeres, present a unique challenge to DNA replication. Due to the nature of lagging strand synthesis, the very end of a linear chromosome cannot be fully replicated.
This leads to a gradual shortening of telomeres with each round of replication. To counteract this, eukaryotic cells employ telomerase, a specialized reverse transcriptase that extends telomeres by adding repetitive DNA sequences.
Telomerase utilizes an internal RNA template to synthesize telomeric DNA, effectively compensating for the shortening that would otherwise occur. Telomere maintenance is crucial for preserving genomic stability and preventing cellular senescence.
The interplay between telomere shortening and telomerase activity is a critical factor in aging and cancer. Dysfunctional telomeres can trigger DNA damage responses and contribute to cellular instability. The structural considerations surrounding telomeres highlight the complexities of replicating linear chromosomes.
Having dissected the intricate enzymatic machinery and the step-by-step progression of DNA replication, it is now prudent to shift our focus to the structural nuances inherent in this fundamental process. The differing mechanisms of leading and lagging strand synthesis, the transient role of RNA primers, and the challenges posed by telomeres underscore the complexity of DNA duplication. Effective communication of these complexities hinges on accurate, clear, and relevant visual representations, particularly the replication fork diagram.
Representing Replication: Accuracy, Clarity, and Relevance
The replication fork diagram stands as a cornerstone in visualizing DNA replication. Its effectiveness, however, depends critically on its accuracy, the level of detail presented, and its overall relevance to broader biological contexts. The diagram must not only capture the core processes but also connect them to their broader implications in heredity and disease.
The Primacy of Accuracy
At its core, any scientific illustration must be accurate. Misrepresentations, even seemingly minor ones, can propagate misunderstandings and impede a true grasp of the underlying biology.
In the context of DNA replication, this means precisely depicting the 5' to 3' directionality of DNA synthesis, the roles of key enzymes, and the distinction between the leading and lagging strands. Shortcuts or oversimplifications that sacrifice accuracy ultimately undermine the educational value of the diagram. Accuracy must be paramount.
Tailoring Detail to the Audience: Level Appropriateness
The level of detail included in a replication fork diagram must be carefully calibrated to the intended audience. A diagram intended for introductory biology students will necessarily differ from one designed for advanced molecular biology researchers.
An overly simplistic diagram may fail to convey the intricacies of the process, while an excessively detailed one can overwhelm and confuse the novice learner. The goal is to strike a balance – to provide sufficient information to facilitate understanding without sacrificing clarity.
Establishing Relevance: Connecting to the Bigger Picture
A replication fork diagram should not exist in isolation. To maximize its impact, it must be connected to the broader context of genetics and its implications for heredity and disease.
This can be achieved by highlighting the consequences of errors in DNA replication, such as mutations that can lead to genetic disorders or cancer. By demonstrating the real-world relevance of DNA replication, the diagram becomes more engaging and meaningful. Understanding the importance fosters deeper engagement.
The Power of Visual Appeal
While accuracy and relevance are essential, the visual appeal of a replication fork diagram should not be overlooked. A well-designed diagram can capture the viewer's attention and make the learning process more enjoyable.
This includes using clear and consistent labeling, employing color strategically to highlight key components, and ensuring that the overall layout is visually pleasing. A visually appealing diagram is more likely to be studied and remembered.
Prioritizing Clarity
Clarity is the ultimate arbiter of a successful replication fork diagram. Even the most accurate and visually appealing diagram will fall short if it is difficult to understand.
This means avoiding jargon, using clear and concise language, and presenting information in a logical and organized manner. The diagram should guide the viewer through the process step-by-step, building understanding incrementally. Clarity of presentation is key.
Subtopics for Clarity include:
- Using Consistent Visual Cues:
- Employing consistent colors and shapes to represent specific enzymes and DNA strands.
- Strategic Use of Arrows and Labels:
- Clearly indicating the direction of replication and the function of each component.
- Minimizing Clutter:
- Avoiding unnecessary details that can distract from the core message.
In conclusion, representing DNA replication effectively requires a multi-faceted approach. Accuracy, level appropriateness, relevance, visual appeal, and clarity must all be carefully considered to create a replication fork diagram that is both informative and engaging. Only then can we truly convey the beauty and complexity of this fundamental process.
Video: Replication Fork Diagram: Guide for Students
FAQs: Replication Fork Diagram
What are the key components always shown in a replication fork diagram?
A replication fork diagram typically includes the origin of replication, the leading strand (synthesized continuously), the lagging strand (synthesized discontinuously in Okazaki fragments), DNA polymerase, helicase, single-strand binding proteins (SSBPs), primase, and DNA ligase. These components work together to duplicate DNA.
Why is the lagging strand synthesized in fragments?
The lagging strand is synthesized in fragments (Okazaki fragments) because DNA polymerase can only add nucleotides to the 3' end of a strand. Since the two strands of DNA are antiparallel, and replication proceeds in one direction, the lagging strand must be synthesized in short, discontinuous segments. The replication fork diagram illustrates this process.
What is the role of helicase in the replication fork diagram?
Helicase is an enzyme that unwinds the double helix of DNA at the replication fork. This unwinding creates a Y-shaped structure, which is represented in the replication fork diagram. Without helicase, DNA would remain tightly bound, and DNA polymerase wouldn't be able to access the strands for replication.
How does a replication fork diagram help in understanding DNA replication?
A replication fork diagram provides a visual representation of the complex processes occurring during DNA replication. By illustrating the roles of various enzymes and the different synthesis patterns of the leading and lagging strands, a replication fork diagram simplifies and clarifies the mechanisms involved in duplicating DNA.
So, there you have it! Hopefully, this guide has demystified the replication fork diagram for you. Don't be afraid to draw it out a few times – practice makes perfect! And remember, understanding this diagram is key to unlocking the secrets of DNA replication. Good luck with your studies!