Photosynthesis Concept Map: Plant Energy Guide
Photosynthesis, a vital process, provides the energy source for plant life, and understanding it thoroughly can be greatly enhanced by visual tools such as a photosynthesis concept map. These maps, similar to those utilized in educational resources developed by Khan Academy, offer a structured overview of the complex reactions involved in converting light energy into chemical energy. Chloroplasts, the organelles within plant cells where photosynthesis occurs, play a pivotal role in this process, utilizing chlorophyll to capture sunlight. Thought leaders like Jan Ingenhousz have significantly contributed to our understanding of photosynthesis through groundbreaking experiments that continue to inform modern botanical studies.
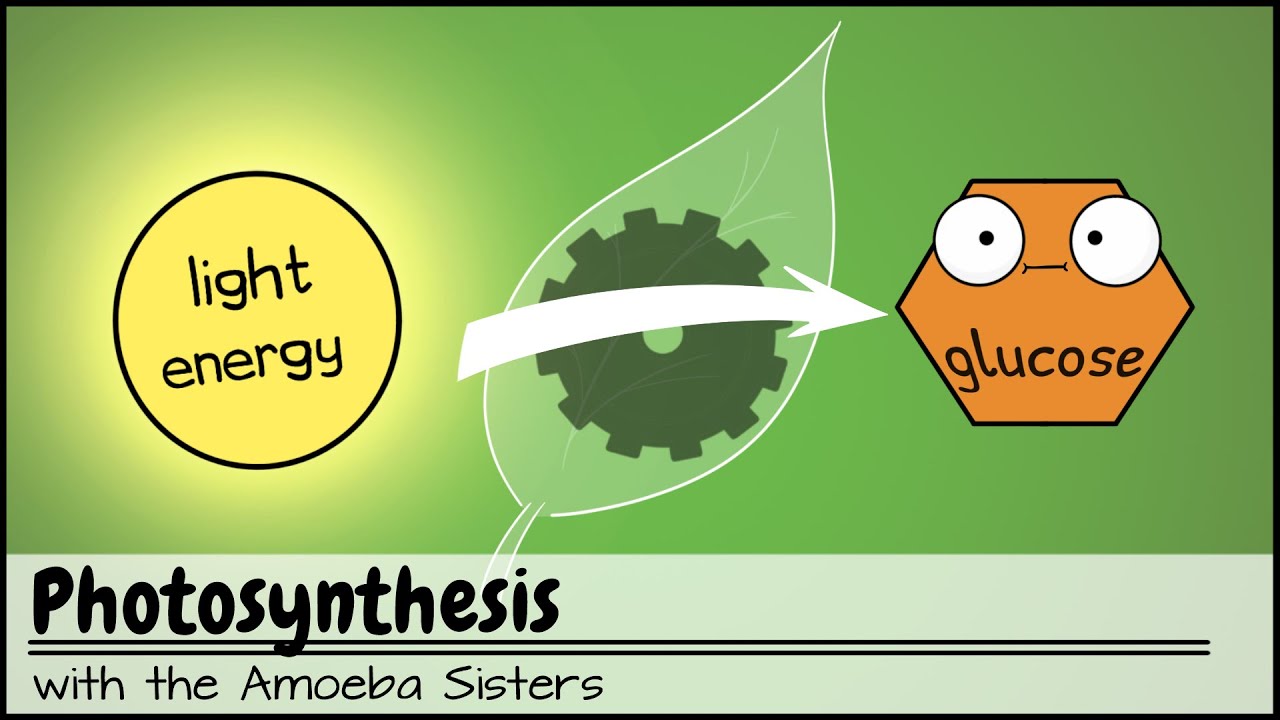
Image taken from the YouTube channel Amoeba Sisters , from the video titled Photosynthesis (UPDATED) .
Photosynthesis is arguably the most important biochemical process on our planet.
It's the elegant mechanism by which plants, algae, and certain bacteria convert light energy into chemical energy, fueling nearly all life on Earth.
This transformative process not only creates the sugars that organisms use for energy, but also releases the oxygen we breathe.
Understanding photosynthesis is crucial for comprehending the intricacies of ecosystems and addressing global challenges like climate change.
The Cornerstone of Life: Autotrophs, Heterotrophs, and Photosynthesis
Photosynthesis forms the base of almost every food chain.
It empowers autotrophs, organisms like plants, to create their own food.
Through photosynthesis, they convert sunlight, water, and carbon dioxide into glucose (a sugar) and oxygen.
Heterotrophs, including animals and fungi, cannot produce their own food.
Instead, they rely on consuming autotrophs or other heterotrophs that have consumed autotrophs.
This dependence highlights the fundamental role of photosynthesis in sustaining the vast majority of life on Earth.
Without it, the energy flow through ecosystems would cease.
A Glimpse into the Past: Early Inquiries into a Vital Process
The journey to understanding photosynthesis has been a long and fascinating one, marked by the contributions of numerous pioneering scientists.
Early investigations, like those of Jan van Helmont, laid the groundwork for unraveling the mysteries of plant nutrition.
Later, scientists like Joseph Priestley observed that plants could "restore" air that had been "injured" by burning candles, unknowingly revealing the oxygen-producing aspect of photosynthesis.
Jan Ingenhousz further refined this understanding by demonstrating that sunlight was essential for this restorative process.
These initial discoveries, though rudimentary by modern standards, set the stage for a deeper exploration of the complex biochemical pathways involved in photosynthesis, leading to the detailed understanding we possess today.
A Glimpse into the Past: Historical Discoveries That Shaped Our Understanding
Photosynthesis is arguably the most important biochemical process on our planet. It's the elegant mechanism by which plants, algae, and certain bacteria convert light energy into chemical energy, fueling nearly all life on Earth. This transformative process not only creates the sugars that organisms use for energy, but also releases the oxygen we breathe. Before we understood the intricate details of photosynthesis, curious minds embarked on groundbreaking experiments. These pivotal moments in scientific history laid the foundation for our current understanding, forever changing how we view the natural world.
Priestley's Pioneering Experiments on Air Purification
Joseph Priestley, an 18th-century English clergyman and scientist, conducted a series of experiments that hinted at the vital role of plants in maintaining the purity of air.
Priestley’s most famous experiment involved placing a burning candle in a closed jar. He observed that the candle quickly extinguished, indicating that the air within the jar had become "injured" or depleted of something essential for combustion and life.
He then introduced a living plant into the same "injured" air. After some time, he found that the air had been restored, allowing a candle to burn once again.
This led him to conclude that plants possess the remarkable ability to purify air, reversing the damage caused by burning or respiration. Priestley had, in essence, stumbled upon the concept of oxygen production, although he didn't yet understand it in those terms.
His observations, though lacking a complete explanation, were a crucial first step in unraveling the mysteries of photosynthesis.
Ingenhousz and the Revelation of Sunlight's Necessity
Jan Ingenhousz, an 18th-century Dutch physiologist, expanded upon Priestley's work, adding a critical piece to the puzzle: sunlight.
Ingenhousz meticulously repeated Priestley's experiments, but he introduced a crucial variable. He observed that plants only purified air when exposed to sunlight.
In the dark, plants had no restorative effect; they even seemed to "injure" the air, similar to a burning candle.
This led Ingenhousz to the revolutionary conclusion that sunlight is not merely a passive element, but an active ingredient in the process of air purification by plants.
He demonstrated that the green parts of plants were responsible for this effect, and that it involved the absorption of "fixed air" (what we now know as carbon dioxide) and the release of "dephlogisticated air" (oxygen).
Ingenhousz’s work provided a more precise understanding of photosynthesis, establishing the critical link between light, plants, and air quality.
The Legacy of Early Discoveries
The experiments of Priestley and Ingenhousz, though conducted with limited knowledge of the underlying biochemical processes, were monumental achievements. They paved the way for future generations of scientists to delve deeper into the intricate mechanisms of photosynthesis.
Their work demonstrated the power of careful observation and experimentation in unraveling the secrets of nature. It also highlights the importance of building upon the work of others, as Ingenhousz's discoveries directly stemmed from Priestley's earlier investigations.
These early pioneers laid the groundwork for our modern understanding of photosynthesis, a process that sustains life as we know it. Their contributions continue to inspire scientific inquiry and underscore the importance of plants in maintaining a healthy planet.
The Two-Step Dance: Light-Dependent and Light-Independent Reactions
Photosynthesis, at its heart, is a carefully choreographed sequence of reactions. Understanding these steps is crucial to grasping the full elegance of this life-sustaining process. It is divided into two major phases: the light-dependent reactions and the light-independent reactions (also known as the Calvin Cycle). Each phase is intricately linked, with the products of one fueling the other, in a seamless conversion of light energy into the chemical energy that sustains life.
Capturing Light's Energy: The Light-Dependent Reactions
The light-dependent reactions occur in the thylakoid membranes of the chloroplasts. This initial phase is all about capturing the energy of sunlight and converting it into a usable form.
Two key protein complexes, Photosystem II (PSII) and Photosystem I (PSI), are at the heart of this process.
When light strikes PSII, electrons are energized and passed along an electron transport chain (ETC). This ETC is a series of protein complexes that facilitate the transfer of electrons.
As electrons move down the ETC, energy is released, which is used to pump protons (H+) across the thylakoid membrane, creating a concentration gradient.
This gradient drives the synthesis of ATP (adenosine triphosphate), an energy-carrying molecule, through a process called chemiosmosis.
Simultaneously, light energy absorbed by PSI re-energizes electrons.
These electrons are then used to reduce NADP+ to NADPH, another energy-carrying molecule.
Crucially, water molecules are split (photolysis) to replenish the electrons lost by PSII. This splitting of water releases oxygen as a byproduct – the very oxygen we breathe.
In essence, the light-dependent reactions convert light energy into chemical energy in the form of ATP and NADPH, and release oxygen.
The Calvin Cycle: Building Sugars from CO2
The light-independent reactions, or the Calvin Cycle, take place in the stroma, the fluid-filled space surrounding the thylakoids in the chloroplast.
This phase uses the ATP and NADPH generated during the light-dependent reactions to fix carbon dioxide and synthesize sugars.
The Calvin Cycle can be broken down into three main stages: carbon fixation, reduction, and regeneration.
-
Carbon Fixation: CO2 from the atmosphere is incorporated into an organic molecule, ribulose-1,5-bisphosphate (RuBP), with the help of the enzyme RuBisCO (ribulose-1,5-bisphosphate carboxylase/oxygenase).
RuBisCO is arguably the most abundant enzyme on Earth.
-
Reduction: The resulting molecule is then reduced using the energy from ATP and the reducing power of NADPH to form glyceraldehyde-3-phosphate (G3P).
G3P is a three-carbon sugar that can be used to build glucose and other organic molecules.
- Regeneration: Finally, RuBP is regenerated so that the cycle can continue. This regeneration also requires ATP.
Powering the Calvin Cycle: The Role of ATP and NADPH
The ATP and NADPH produced during the light-dependent reactions are the driving force behind the Calvin Cycle.
ATP provides the energy needed for several steps in the cycle, including the reduction of the initial carbon fixation product and the regeneration of RuBP.
NADPH provides the reducing power, donating electrons to convert the initial carbon fixation product into G3P.
Without a continuous supply of ATP and NADPH from the light-dependent reactions, the Calvin Cycle would grind to a halt, and the plant would be unable to produce the sugars it needs to survive.
In conclusion, the two-step dance of photosynthesis – the light-dependent and light-independent reactions – exemplifies the elegant efficiency of nature.
The light-dependent reactions capture light energy and convert it into chemical energy, while the Calvin Cycle uses that energy to build sugars from carbon dioxide.
Understanding this intricate interplay is key to appreciating the fundamental role of photosynthesis in sustaining life on Earth.
Key Players: Chlorophyll, Chloroplasts, and Other Essential Molecules
Photosynthesis, at its heart, is a carefully choreographed sequence of reactions. Understanding these steps is crucial to grasping the full elegance of this life-sustaining process. It relies on specialized molecules and structures to capture light energy and convert it into chemical energy.
At the forefront of these key players are chlorophyll, the green pigment that gives plants their characteristic color, and the chloroplast, the organelle where photosynthesis unfolds. These elements work in concert to perform one of the most vital processes on our planet.
Chlorophyll: Capturing the Sun's Energy
Chlorophyll is not just a pigment; it's the primary light-capturing molecule in plants, algae, and cyanobacteria. Its structure, a porphyrin ring with a magnesium ion at its center, allows it to absorb specific wavelengths of light from the visible spectrum.
This absorption is the first step in photosynthesis, initiating the chain of events that ultimately convert light energy into chemical energy. Chlorophyll absorbs blue and red light most efficiently, reflecting green light, which is why plants appear green to our eyes.
There are several types of chlorophyll (chlorophyll a, chlorophyll b, etc.), each with slightly different absorption spectra, allowing plants to capture a broader range of light. These subtle differences are essential for maximizing photosynthetic efficiency in various environmental conditions.
The Chloroplast: The Photosynthetic Powerhouse
The chloroplast is the organelle within plant cells where photosynthesis takes place. It’s a complex structure, housing all the necessary machinery for both the light-dependent and light-independent reactions. Understanding its architecture is key to understanding how photosynthesis works.
Thylakoid Membranes: Where Light Reactions Occur
Within the chloroplast are thylakoids, flattened, sac-like membranes arranged in stacks called grana (singular: granum). These membranes are the site of the light-dependent reactions.
Embedded in the thylakoid membranes are chlorophyll molecules and other pigment proteins, organized into photosystems. Photosystems I and II capture light energy and initiate the electron transport chain, leading to the production of ATP and NADPH, the energy currencies of the cell.
The thylakoid membrane also creates a proton gradient, essential for ATP synthesis. This intricate system of proteins and pigments ensures efficient energy capture and conversion.
Grana and Stroma: Organization for Efficiency
The grana, stacks of thylakoids, maximize the surface area available for light capture. The stroma, the fluid-filled space surrounding the thylakoids, is where the light-independent reactions (Calvin Cycle) take place.
This spatial separation of the two phases of photosynthesis allows for efficient coordination. The ATP and NADPH produced in the thylakoids are readily available in the stroma to power the Calvin Cycle.
The stroma also contains the enzymes needed for carbon fixation, including RuBisCO, the enzyme that catalyzes the first major step of the Calvin Cycle.
The chloroplast, with its carefully organized structures, is a masterpiece of biological engineering, enabling plants to harness the power of the sun and sustain life on Earth.
Pathways to Success: Exploring C3, C4, and CAM Photosynthesis
Photosynthesis, at its heart, is a carefully choreographed sequence of reactions. Understanding these steps is crucial to grasping the full elegance of this life-sustaining process. It relies on specialized molecules and structures to capture light energy and convert it into chemical energy. But the path isn't always the same. Plants, masters of adaptation, have evolved diverse photosynthetic strategies to thrive in a variety of environments. Let's delve into the fascinating world of C3, C4, and CAM photosynthesis.
C3 Photosynthesis: The Standard Pathway
C3 photosynthesis represents the most common and ancestral pathway for carbon fixation in plants. It's the workhorse of the plant kingdom, found in familiar crops like rice, wheat, and soybeans, as well as many trees and other vegetation. The "C3" designation stems from the initial product of carbon fixation. Carbon dioxide directly combines with ribulose-1,5-bisphosphate (RuBP). This reaction is catalyzed by the enzyme RuBisCO, forming a three-carbon compound: 3-phosphoglycerate (3-PGA).
This initial step occurs within the mesophyll cells of the leaf. Subsequently, 3-PGA undergoes a series of biochemical reactions within the Calvin cycle. This produces sugars and regenerates RuBP, allowing the cycle to continue.
Drawbacks of C3 Photosynthesis: Photorespiration
However, C3 photosynthesis is not without its limitations. RuBisCO, the enzyme responsible for carbon fixation, also has an affinity for oxygen. In hot, dry conditions, plants close their stomata to conserve water, leading to a buildup of oxygen and a decrease in carbon dioxide within the leaf.
When oxygen binds to RuBisCO instead of carbon dioxide, a process called photorespiration occurs.
Photorespiration is a metabolically costly process that consumes energy and releases carbon dioxide. This reduces the overall efficiency of photosynthesis, especially in warm climates.
C4 Photosynthesis: An Adaptation to Warm Climates
C4 photosynthesis represents a remarkable adaptation to overcome the limitations of C3 photosynthesis in hot, arid environments. Plants employing this pathway, such as corn, sugarcane, and sorghum, have evolved a specialized leaf anatomy. This anatomy helps to minimize photorespiration and enhance carbon fixation.
The C4 Pathway: A Two-Step Process
In C4 plants, carbon dioxide is initially fixed in the mesophyll cells by an enzyme called PEP carboxylase. PEP carboxylase has a much higher affinity for carbon dioxide than RuBisCO, and does not bind to oxygen. The product of this reaction is a four-carbon compound, oxaloacetate (hence the "C4").
Oxaloacetate is then converted to malate or aspartate and transported to specialized bundle sheath cells surrounding the vascular bundles of the leaf. Within the bundle sheath cells, the four-carbon compound is decarboxylated. This releases carbon dioxide, which is then fixed by RuBisCO in the Calvin cycle, as in C3 plants.
By concentrating carbon dioxide in the bundle sheath cells, C4 photosynthesis minimizes photorespiration. This makes it more efficient than C3 photosynthesis in hot, dry conditions where stomata are closed.
CAM Photosynthesis: Surviving Extreme Drought
Crassulacean Acid Metabolism (CAM) photosynthesis represents an even more specialized adaptation to extreme drought conditions. This pathway is found in succulent plants like cacti, pineapples, and sedums, which thrive in arid and semi-arid environments.
CAM: Temporal Separation of Carbon Fixation
CAM plants take water conservation to the extreme. Unlike C3 and C4 plants, CAM plants perform carbon fixation and the Calvin cycle at different times of the day. At night, when temperatures are cooler and humidity is higher, CAM plants open their stomata and take in carbon dioxide.
The carbon dioxide is fixed by PEP carboxylase. This yields oxaloacetate, which is converted to malate and stored in vacuoles within the mesophyll cells. During the day, when the stomata are closed to conserve water, the malate is decarboxylated, releasing carbon dioxide. The carbon dioxide is then fixed by RuBisCO in the Calvin cycle.
This temporal separation of carbon fixation and the Calvin cycle allows CAM plants to minimize water loss while still carrying out photosynthesis, making them well-suited to survive in harsh, dry environments.
Comparing C3, C4, and CAM: A Trade-Off
Each photosynthetic pathway represents a unique adaptation to specific environmental conditions. C3 photosynthesis is the most common but is less efficient in hot, dry climates due to photorespiration. C4 photosynthesis minimizes photorespiration and is more efficient in warm environments. CAM photosynthesis maximizes water conservation in extremely arid conditions.
The choice of photosynthetic pathway involves a trade-off between efficiency, water conservation, and energy expenditure. Understanding these pathways is crucial for comprehending plant adaptation. Understanding these pathways helps us address challenges in agriculture and climate change.
Factors Influencing Photosynthesis: Light, CO2, and Water
Pathways to Success: Exploring C3, C4, and CAM Photosynthesis Photosynthesis, at its heart, is a carefully choreographed sequence of reactions. Understanding these steps is crucial to grasping the full elegance of this life-sustaining process. It relies on specialized molecules and structures to capture light energy and convert it into chemical energy. However, the efficiency of this process isn't constant; it's influenced by several environmental factors.
Like any complex system, photosynthesis is subject to limitations. Three key factors—light intensity, carbon dioxide concentration, and water availability—can significantly impact its rate. Understanding these limitations is crucial not just for scientific understanding, but for optimizing plant growth in agriculture and beyond.
The Law of Limiting Factors
The concept of limiting factors in photosynthesis underscores that the rate of the process is governed by the factor that is in shortest supply. This principle, often referred to as Liebig's Law of the Minimum, dictates that even if all other factors are optimal, the rate of photosynthesis will be constrained by the most deficient resource.
Light Intensity: The Prime Driver
Light is the initial energy source for photosynthesis, and its intensity directly affects the rate of the light-dependent reactions. At low light intensities, the rate of photosynthesis is limited because there isn't enough energy to drive the electron transport chain.
As light intensity increases, the rate of photosynthesis generally increases proportionally—up to a point. Beyond a certain threshold, the photosynthetic machinery becomes saturated, and further increases in light intensity do not lead to a corresponding increase in the rate of photosynthesis. In fact, excessively high light can even damage the photosynthetic apparatus, a phenomenon known as photoinhibition.
Carbon Dioxide Concentration: The Carbon Source
Carbon dioxide (CO2) is the essential building block for sugar synthesis in the Calvin cycle. As such, its concentration around the plant plays a critical role in determining the rate of photosynthesis.
In many natural environments, CO2 concentration is often a limiting factor, especially on hot, dry days when plants close their stomata to conserve water. When CO2 levels are low, the enzyme RuBisCO, responsible for carbon fixation, struggles to function effectively, slowing down the entire Calvin cycle.
Interestingly, research suggests that increasing CO2 concentration in the atmosphere (within reasonable limits) can enhance photosynthetic rates in some plants, potentially boosting agricultural yields. However, this benefit needs to be weighed against the broader environmental impacts of elevated CO2 levels.
Water Availability: The Essential Solvent and Coolant
Water is not only essential as a reactant in photosynthesis but also plays a critical role in maintaining plant turgor pressure and facilitating gas exchange through the stomata. Water scarcity can severely limit photosynthesis in several ways.
First, a lack of water causes the stomata to close, restricting the entry of CO2 into the leaf. This, in turn, reduces the availability of CO2 for the Calvin cycle.
Second, water stress can directly impact the photosynthetic machinery, damaging enzymes and reducing the efficiency of electron transport. In extreme cases, water stress can lead to wilting and even death of the plant.
The Role of Stomata: Gatekeepers of Gas Exchange
Stomata are microscopic pores on the surface of leaves that regulate the exchange of gases (CO2 and oxygen) between the plant and the atmosphere. They also control water loss through transpiration.
The opening and closing of stomata are carefully regulated by environmental factors, such as light, CO2 concentration, and water availability. When water is scarce, plants close their stomata to conserve water, but this also limits CO2 uptake, thereby reducing the rate of photosynthesis. Conversely, when water is plentiful, stomata remain open, allowing for efficient gas exchange.
Implications for Agriculture
Understanding the limiting factors of photosynthesis has profound implications for agriculture. By carefully managing light intensity, CO2 concentration, and water availability, farmers can optimize plant growth and productivity.
For example, in greenhouses, supplemental lighting and CO2 enrichment can be used to enhance photosynthetic rates, leading to increased yields. Similarly, irrigation systems can ensure that plants receive adequate water, even during periods of drought.
Furthermore, selecting crop varieties that are adapted to specific environmental conditions is crucial for maximizing photosynthetic efficiency. For instance, C4 plants, which are more efficient at fixing CO2 under low concentrations, are often favored in hot, dry climates.
By understanding and addressing the limiting factors of photosynthesis, we can enhance agricultural productivity and ensure food security for a growing global population. Moreover, insights gained from studying photosynthesis can also be applied to develop sustainable energy solutions, such as artificial photosynthesis, which aims to mimic the natural process to produce clean fuels.
Photosynthesis and the Environment: A Crucial Connection
Photosynthesis, at its heart, is a carefully choreographed sequence of reactions. Understanding these steps is crucial to grasping the full elegance of this life-sustaining process. It relies on specialized molecules and structures that transform light energy into the very building blocks of life. But the story of photosynthesis extends far beyond the individual plant, intertwining with the health and stability of our entire planet.
The Interplay Between Photosynthesis and Cellular Respiration
Photosynthesis and cellular respiration are two sides of the same coin. These two processes are intimately linked in a cycle of energy conversion and exchange. Photosynthesis captures light energy and uses it to create sugars (glucose) from carbon dioxide and water.
Cellular respiration, on the other hand, breaks down these sugars, releasing the stored energy to fuel the plant's growth, development, and other life processes. The byproducts of cellular respiration – carbon dioxide and water – are then used again in photosynthesis, completing the cycle.
Photosynthesis as the Foundation of Plant Life
The glucose produced during photosynthesis is not merely a source of energy. It is also the fundamental building block for plant biomass. Plants use glucose to synthesize cellulose, the main component of cell walls. They also create starches for energy storage, and a myriad of other organic compounds essential for their survival.
Without photosynthesis, plants would lack both the energy and the structural materials needed to grow and thrive. Ultimately, every part of the plant – its leaves, stems, roots, and flowers – owes its existence to this remarkable process.
The Carbon Dioxide Balancing Act
One of the most significant environmental roles of photosynthesis is its ability to remove carbon dioxide (CO2) from the atmosphere. CO2 is a greenhouse gas that traps heat and contributes to climate change. By absorbing CO2 during photosynthesis, plants help to regulate the Earth's temperature and maintain a stable climate.
This is not just a theoretical concept; it has profound implications for our planet's future. As atmospheric CO2 levels continue to rise, the importance of photosynthesis in mitigating climate change becomes ever more critical.
Carbon Sequestration: Harnessing Photosynthesis for a Sustainable Future
Carbon sequestration refers to the long-term storage of carbon in plants, soils, and oceans. Photosynthesis plays a central role in this process. Through photosynthesis, plants capture CO2 and convert it into organic matter, effectively locking away carbon in their tissues.
Forests, grasslands, and other ecosystems act as major carbon sinks, absorbing vast amounts of CO2 from the atmosphere. Protecting and restoring these ecosystems is essential for enhancing carbon sequestration and reducing greenhouse gas emissions. Furthermore, scientists are exploring innovative ways to enhance photosynthetic efficiency in crops and forests. The goal is to maximize carbon capture and contribute to a more sustainable future.
Energy Flow and Biomass: A Sustainable Ecosystem
Photosynthesis is the entry point of energy into most ecosystems. The energy captured by plants is then transferred to other organisms through the food chain. Herbivores consume plants, carnivores consume herbivores, and so on. This continuous transfer of energy sustains the entire ecosystem.
The total mass of living organisms in a given area, known as biomass, is directly related to the rate of photosynthesis. Ecosystems with high photosynthetic activity tend to have larger biomass, supporting a greater diversity of life. Understanding the relationship between photosynthesis, energy flow, and biomass is crucial for managing and conserving our planet's ecosystems. It allows us to make informed decisions about land use, resource management, and climate change mitigation.
Tools and Techniques: Unraveling the Mysteries of Photosynthesis
Photosynthesis, at its heart, is a carefully choreographed sequence of reactions. Understanding these steps is crucial to grasping the full elegance of this life-sustaining process. It relies on specialized molecules and structures that transform light energy into the very building blocks of life. But how do scientists peek inside this miniature, sun-powered factory? A suite of ingenious tools and techniques allow researchers to dissect the process, revealing its secrets at the molecular level.
Spectrophotometry: Measuring Light's Dance
Spectrophotometry stands as a cornerstone technique for understanding how plants capture light. At its core, spectrophotometry quantifies the absorption and transmission of light through a substance. This is particularly valuable in photosynthesis research, as it allows scientists to analyze how pigments like chlorophyll absorb different wavelengths of light.
By shining a beam of light through a sample of chlorophyll extract, for example, and measuring the amount of light that passes through, researchers can determine the specific wavelengths absorbed by the pigment. This information reveals which parts of the light spectrum are most effective in driving photosynthesis. This is crucial for understanding energy absorption efficiency.
Gas Exchange Measurements: Tracking Carbon Dioxide and Oxygen
Photosynthesis is a process of gas exchange, with plants consuming carbon dioxide and releasing oxygen. Gas exchange measurements provide a direct way to monitor this exchange, offering insights into the overall rate of photosynthesis. These measurements involve enclosing a plant or leaf in a controlled chamber and monitoring the changes in carbon dioxide and oxygen concentrations over time.
By carefully controlling the conditions within the chamber, such as light intensity, temperature, and humidity, researchers can assess how these factors influence the rate of photosynthesis. For example, a sudden increase in light intensity might lead to a corresponding increase in carbon dioxide uptake, indicating that light is a limiting factor.
Understanding this interplay is crucial for optimizing plant growth in various environments. Sophisticated instruments can now track these gas fluxes in real-time.
Microscopy: Visualizing the Chloroplast's Architecture
Microscopy offers a visual window into the intricate world of the chloroplast, the organelle where photosynthesis takes place. Light microscopy allows scientists to observe the overall structure of chloroplasts within plant cells, revealing the arrangement of thylakoids and grana.
Electron microscopy, with its much higher resolution, provides even more detailed views, revealing the fine structure of the thylakoid membranes and the location of key photosynthetic proteins. This is invaluable for understanding how the architecture of the chloroplast facilitates the light-dependent reactions.
Radioactive Tracers: Following Carbon's Journey
Radioactive tracers provide a powerful way to track the path of carbon as it moves through the photosynthetic pathway. By providing plants with carbon dioxide labeled with a radioactive isotope of carbon (14C), researchers can follow the movement of this carbon atom through the various steps of the Calvin cycle.
This technique allowed Melvin Calvin and his colleagues to map the entire cycle, identifying the intermediate compounds formed during carbon fixation and reduction. The use of radioactive tracers continues to be a valuable tool for elucidating complex metabolic pathways.
Concept Mapping Software: Visualizing Complex Processes
While not a direct experimental technique, concept mapping software plays a critical role in synthesizing and visualizing the vast amount of data generated by photosynthesis research. These tools allow scientists to create diagrams that illustrate the relationships between different concepts and processes.
By visually representing the complex interactions within the photosynthetic pathway, concept maps can help researchers identify knowledge gaps, generate new hypotheses, and communicate their findings more effectively. Concept mapping becomes a powerful tool to structure the mental frameworks of photosynthesis, translating complexity into comprehension.
Contemporary Research and Resources: Staying Updated on Photosynthesis
Photosynthesis, at its heart, is a carefully choreographed sequence of reactions. Understanding these steps is crucial to grasping the full elegance of this life-sustaining process. It relies on specialized molecules and structures that transform light energy into the very building blocks of life as we know it. But our knowledge of photosynthesis isn't static; it’s a continuously evolving field, propelled by groundbreaking research and accessible resources.
The Legacy of Calvin and Benson
The mid-20th century saw a monumental leap in our understanding of photosynthesis with the work of Melvin Calvin and Andrew Benson. Their meticulous research led to the complete mapping of the Calvin Cycle, the light-independent reactions where carbon dioxide is fixed into sugars.
Using radioactive carbon-14 as a tracer, they illuminated the intricate pathways of carbon assimilation, revealing the key enzymes and intermediate compounds involved.
This Nobel Prize-winning work provided the foundation for much of what we know about carbon fixation today, paving the way for future explorations into enhancing photosynthetic efficiency.
Modern Pioneers in Photosynthesis Research
While Calvin and Benson laid the groundwork, contemporary plant biologists and biochemists are building upon their legacy, pushing the boundaries of our understanding of photosynthesis.
Researchers are actively investigating the complexities of photosystems, exploring ways to optimize light capture and energy transfer.
Others are focused on enhancing the efficiency of RuBisCO, the enzyme responsible for carbon fixation, which is notoriously inefficient.
These endeavors hold immense potential for improving crop yields and addressing global food security.
Furthermore, scientists are delving into the genetic and molecular mechanisms that regulate photosynthesis, seeking to engineer plants with improved photosynthetic performance under various environmental stresses.
This includes research into manipulating gene expression to enhance drought tolerance, increase carbon dioxide uptake, and improve nutrient use efficiency.
Accessible Educational Resources for Aspiring Photosynthesis Experts
The wealth of knowledge surrounding photosynthesis is no longer confined to research labs and academic journals.
Numerous online learning platforms offer accessible educational content on this vital process, catering to a wide range of learners.
- Coursera: Platforms such as Coursera host courses from leading universities, providing in-depth explorations of photosynthesis.
- edX: edX offers similar opportunities to learn from experts in the field through structured online courses.
- Khan Academy: For a more introductory approach, Khan Academy provides excellent free resources that break down the fundamentals of photosynthesis.
- University Websites: Many universities offer open access to lecture materials and research publications, allowing anyone to delve into the latest findings in photosynthesis research.
These resources empower individuals to expand their knowledge, engage with cutting-edge research, and contribute to a deeper understanding of the engine that drives life on Earth.
Staying updated is critical, as researchers are constantly making breakthroughs that reshape our understanding and unlock new possibilities.
Video: Photosynthesis Concept Map: Plant Energy Guide
FAQs: Photosynthesis Concept Map - Plant Energy Guide
What's the main purpose of a photosynthesis concept map?
A photosynthesis concept map visually organizes the key processes and components involved in how plants convert light energy into chemical energy. It helps you understand the relationships between elements like sunlight, water, carbon dioxide, glucose, and oxygen.
How does the photosynthesis concept map show the role of sunlight?
The concept map highlights that sunlight is the primary energy source. Chlorophyll in chloroplasts absorbs this light energy, which fuels the conversion of water and carbon dioxide into glucose (sugar) – the plant's food.
Where does carbon dioxide come into play in the photosynthesis concept map?
Carbon dioxide (CO2) is a critical reactant. The photosynthesis concept map illustrates how plants absorb CO2 from the atmosphere. This CO2, along with water, is used during the light-independent reactions (Calvin cycle) to produce glucose.
What does a photosynthesis concept map reveal about oxygen?
The photosynthesis concept map clearly shows oxygen (O2) as a byproduct of photosynthesis. It's released back into the atmosphere after the plant splits water molecules during the light-dependent reactions, making it essential for life on Earth.
So, there you have it! Hopefully, this journey through photosynthesis has shed some light on how plants fuel their lives. Now, go forth and create your own killer photosynthesis concept map – it's a fantastic way to really solidify your understanding of this amazing process. Happy learning!