Decoding the Oxygen Phase Diagram: The Ultimate Guide
Understanding the behavior of oxygen under varying conditions is critical in numerous scientific and industrial applications. The University of Cambridge studies materials science extensively, and their research contributes significantly to our understanding of the oxygen phase diagram. The Clausius-Clapeyron relation provides a theoretical framework, and its application helps scientists predict phase transitions within the oxygen phase diagram. Exploring the complexities of solid, liquid, and gaseous oxygen phases often requires specialized tools, such as X-ray diffraction, for precise analysis. The National Institute of Standards and Technology (NIST) provides crucial reference data, including thermodynamic properties essential for accurately interpreting the oxygen phase diagram. Therefore, a thorough examination of the oxygen phase diagram reveals the intricate relationships between temperature, pressure, and the resulting physical state of oxygen, insights valuable for fields ranging from medicine to materials engineering.
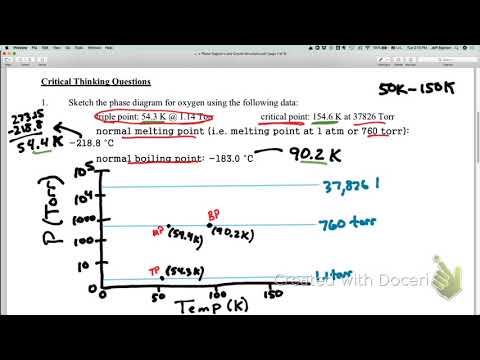
Image taken from the YouTube channel Jeffrey Sigman , from the video titled Phase Diagram O2 - Rough Drawing .
Unveiling the Mysteries of Oxygen's Phases
Oxygen. The very air we breathe, the lifeblood of countless biological and industrial processes. We often perceive it as a simple, ubiquitous element. However, beneath this veneer of simplicity lies a surprisingly complex world of phases and transformations.
Its phase diagram, a map of its existence under varying conditions, reveals a landscape far richer and more intricate than one might expect.
Phase Diagrams: Roadmaps of Material Behavior
Phase diagrams are the cornerstone of materials science. They are graphical representations illustrating the thermodynamically stable phases of a substance under different conditions, typically temperature and pressure.
These diagrams are essential tools for predicting and controlling material behavior in a wide array of applications. They allow scientists and engineers to determine the optimal conditions for processing materials, designing new alloys, and understanding the behavior of substances under extreme conditions.
Essentially, they act as roadmaps, guiding us through the complex world of material transformations.
Oxygen: A Case Study in Phasal Complexity
While many substances exhibit relatively simple phase diagrams, oxygen stands out due to its remarkable diversity of solid phases. Under increasing pressure and decreasing temperature, oxygen transforms into a series of distinct solid structures, each with unique properties.
These solid phases exhibit a fascinating range of behaviors, including changes in color, magnetic ordering, and even the emergence of superconductivity under extreme conditions. The study of these phases provides valuable insights into the fundamental nature of chemical bonding, intermolecular interactions, and the behavior of matter under extreme conditions.
The unusual behavior of oxygen stems from its electronic structure and intermolecular interactions, making it a compelling subject for both experimental and theoretical investigations.
Exploring the Oxygen Phase Diagram: An Overview
This article will delve into the fascinating world of the oxygen phase diagram, exploring the various phases of oxygen. We will examine its properties under different conditions of temperature and pressure.
We will investigate the underlying scientific principles that govern its behavior. We will also discuss the practical applications of understanding oxygen's phase diagram across various scientific and industrial fields.
Prepare to journey into the heart of matter, where the seemingly simple element of oxygen reveals a world of unexpected complexity and scientific intrigue.
Phase diagrams reveal the fascinating interplay between a substance’s physical state and the conditions it experiences. Before we can explore the complexities of oxygen’s phasal landscape, it is essential to establish a solid foundation in the fundamentals of phase diagrams themselves. Consider this section a primer, designed to equip you with the knowledge necessary to navigate these informative graphical representations.
Phase Diagram Fundamentals: A Primer
At its core, a phase diagram is a visual representation of the thermodynamically stable phases of a substance under varying conditions. Understanding these diagrams requires familiarity with the definition of a "phase," the significance of the axes, and the interpretation of key features such as phase boundaries, triple points, and critical points.
Defining a "Phase"
In the context of thermodynamics, a phase refers to a physically distinct and chemically homogeneous region of a system. It has uniform physical properties and is separated from other phases by distinct boundaries. The three most commonly encountered phases are:
- Solid: Characterized by a fixed shape and volume due to strong intermolecular forces.
- Liquid: Possessing a fixed volume but adapting its shape to fit its container, exhibiting weaker intermolecular forces than solids.
- Gas: Exhibiting neither a fixed shape nor a fixed volume, expanding to fill available space due to very weak intermolecular forces.
Some substances can exhibit multiple solid phases, each with a unique crystal structure and properties, as we'll see with oxygen.
Understanding the Axes: Temperature and Pressure
Phase diagrams typically plot temperature on the x-axis and pressure on the y-axis.
Temperature represents the average kinetic energy of the molecules within the substance.
Pressure reflects the force exerted per unit area on the substance.
By mapping the phase stability under different temperatures and pressures, the diagram reveals which phase or phases are thermodynamically favored under any given set of conditions.
Key Features of a Phase Diagram
Phase Boundaries
Phase boundaries are lines on the diagram that represent the conditions under which two or more phases can coexist in equilibrium.
Crossing a phase boundary indicates a phase transition, where the substance changes from one phase to another (e.g., melting, boiling, sublimation).
The slope and shape of these boundaries provide valuable information about the thermodynamics of the phase transition.
The Triple Point
The triple point is a unique point on the phase diagram where all three phases (solid, liquid, and gas) coexist in thermodynamic equilibrium.
It represents a specific temperature and pressure at which the substance can exist simultaneously in all three states.
The triple point is an invariant point, meaning its temperature and pressure are uniquely defined for a given substance.
The Critical Point
At the critical point, the distinction between the liquid and gas phases disappears.
Beyond this point, the substance exists as a supercritical fluid, a state of matter with properties intermediate between those of a liquid and a gas.
The critical point is characterized by a critical temperature (Tc) and a critical pressure (Pc).
Above Tc, the substance cannot be liquefied by increasing pressure alone.
Overview of Phase Transitions
Phase transitions are physical processes that involve a change in the phase of a substance due to changes in temperature, pressure, or both.
Common examples include:
- Melting: Solid to liquid.
- Freezing: Liquid to solid.
- Boiling (Vaporization): Liquid to gas.
- Condensation: Gas to liquid.
- Sublimation: Solid to gas.
- Deposition: Gas to solid.
Understanding the nature of these transitions and the conditions under which they occur is crucial for interpreting phase diagrams.
Phase diagrams reveal the fascinating interplay between a substance’s physical state and the conditions it experiences. Before we can explore the complexities of oxygen’s phasal landscape, it is essential to establish a solid foundation in the fundamentals of phase diagrams themselves. Consider this section a primer, designed to equip you with the knowledge necessary to navigate these informative graphical representations.
Decoding the Oxygen Phase Diagram: A Detailed Exploration
Having armed ourselves with the fundamental principles of phase diagrams, we can now embark on a journey into the intricate world of oxygen's phases. The oxygen phase diagram is not a simple affair; it is a rich tapestry of solid, liquid, and gaseous states, each with its unique characteristics and behaviors under specific conditions. What sets oxygen apart is its stunning variety of solid phases, each emerging under different pressure and temperature regimes.
An Overview of the Oxygen Phase Diagram
The oxygen phase diagram maps out the stable phases of oxygen across a wide range of temperatures and pressures. At low pressures and ambient temperatures, oxygen exists as a gas. As temperature decreases, it transitions into a liquid. However, the most fascinating aspect lies in its solid phases, which appear under varying pressure conditions.
These solid phases, denoted by Greek letters (alpha, beta, gamma, delta, epsilon, zeta, eta, and theta), exhibit distinct crystal structures and physical properties. Understanding this diagram provides critical insights into oxygen's behavior under diverse conditions.
The Gaseous Oxygen Phase
At standard atmospheric pressure and room temperature, oxygen exists as a diatomic gas ($O
_2$). This gaseous phase is essential for respiration and combustion processes.
Behavior at Different Temperatures and Pressures
The behavior of gaseous oxygen is largely governed by the ideal gas law at lower pressures. As pressure increases, deviations from ideality become more pronounced due to intermolecular interactions.
Temperature plays a crucial role; increasing the temperature enhances the kinetic energy of the molecules, promoting expansion, while decreasing temperature reduces kinetic energy.
Transition to Liquid
Upon cooling to its boiling point (-183°C or 90K at 1 atm), gaseous oxygen transitions into liquid oxygen. This phase change involves a significant decrease in volume and an increase in density as the molecules come closer together.
Exploring the Liquid Oxygen Phase
Liquid oxygen ($LOX$) is a pale blue, cryogenic liquid with unique properties that make it useful in various applications.
Properties and Behavior
Liquid oxygen is a cryogenic fluid with a high density compared to its gaseous counterpart. It is paramagnetic due to the unpaired electrons in the $O_2$ molecule.
Liquid oxygen is also a powerful oxidizing agent and must be handled with care to avoid combustion hazards.
Applications
Liquid oxygen finds widespread use as an oxidizer in rocket propulsion systems. It is also employed in industrial processes, such as steelmaking and in medical applications, like oxygen therapy for patients with respiratory issues.
Transition to Solid Phases
Cooling liquid oxygen further leads to solidification. However, unlike many substances that directly form a single solid phase, oxygen exhibits a series of solid phases depending on the applied pressure. This is where the oxygen phase diagram reveals its true complexity.
Delving into the Solid Oxygen Phases
The solid phases of oxygen are where its phase diagram truly shines, displaying a range of structural arrangements dictated by temperature and pressure.
Introducing the Solid Phases
Solid oxygen is a fascinating example of polymorphism, where a single element exists in multiple crystalline forms. These forms, typically denoted α, β, γ, δ, ε, ζ, η, and θ, are stable under different pressure and temperature conditions.
Detailed Descriptions of Each Phase
-
Alpha Phase (α-$O
_2$)
: This is the stable phase at low temperatures and pressures. It features a monoclinic crystal structure with antiferromagnetic ordering. It exists at temperatures below 23.65 K at ambient pressure. -
Beta Phase (β-$O_2$): As temperature increases, oxygen transforms into the beta phase, characterized by a rhombohedral crystal structure. The beta phase is stable between 23.65 K and 43.8 K at ambient pressure.
-
Gamma Phase (γ-$O
_2$)
: Further increasing temperature leads to the gamma phase, with a cubic crystal structure. It's stable between 43.8 K and its melting point. It is unique for exhibiting molecular rotation. -
Delta Phase (δ-$O_2$): At higher pressures, oxygen forms the delta phase, with a more complex crystal structure.
-
Epsilon Phase (ε-$O2$): The epsilon phase, also known as red oxygen, emerges at pressures above 10 GPa and room temperature. It exhibits a unique $O4$ tetramer structure and interesting magnetic properties.
-
Zeta Phase (ζ-$O
_2$)
: At even higher pressures, above 96 GPa, the zeta phase appears. -
Eta Phase (η-$O_2$): This metallic phase forms at extremely high pressures (above 100 GPa). It displays superconductivity at low temperatures, an extraordinary behavior for oxygen.
-
Theta Phase (θ-$O_2$): The theta phase is another high-pressure metallic phase of oxygen.
Factors Influencing the Formation of Each Phase
The formation of these various solid phases is influenced by several factors:
-
Pressure: High pressure forces the oxygen molecules closer together, leading to different crystal structures.
-
Temperature: Temperature affects the kinetic energy of the molecules and influences the stability of different phases.
-
Intermolecular Interactions: The balance between attractive and repulsive forces between oxygen molecules plays a critical role in determining the crystal structure.
Unusual Properties: Superconductivity and Magnetism
The high-pressure phases of oxygen exhibit remarkable properties, including superconductivity and magnetism. The metallic eta phase becomes superconducting at low temperatures, offering insights into the fundamental nature of superconductivity. Furthermore, many solid oxygen phases exhibit intriguing magnetic behaviors, such as antiferromagnetism, due to the electron spin of the oxygen molecules. These properties make solid oxygen a fascinating subject for condensed matter physics research.
Having armed ourselves with the fundamental principles of phase diagrams, we can now embark on a journey into the intricate world of oxygen's phases. The oxygen phase diagram is not a simple affair; it is a rich tapestry of solid, liquid, and gaseous states, each with its unique characteristics and behaviors under specific conditions. What sets oxygen apart is its stunning variety of solid phases, each emerging under different pressure and temperature regimes.
The Science Behind Oxygen's Phases: Thermodynamics and Modeling
The complex phase behavior of oxygen arises from the interplay of fundamental scientific principles. Thermodynamics dictates the stability of phases under varying conditions. Quantum mechanics explains the nature of chemical bonds within oxygen molecules and solids. Statistical mechanics provides a framework for understanding the collective behavior of large ensembles of oxygen atoms. Finally, Density Functional Theory (DFT) offers a powerful computational tool for modeling and predicting phase behavior.
Thermodynamics and Phase Transitions
Thermodynamics provides the bedrock for understanding phase stability. The key concept is that a system will tend towards the state with the lowest Gibbs free energy. This energy depends on the system's enthalpy (related to internal energy and pressure) and entropy (related to disorder).
Temperature and pressure directly influence a phase's Gibbs free energy. For example, increasing temperature favors phases with higher entropy (like gases). Increasing pressure favors phases with lower volume (typically solids).
The Clausius-Clapeyron Equation
The Clausius-Clapeyron equation is a powerful tool for predicting the slope of phase boundaries on a phase diagram. This equation mathematically relates the slope to the enthalpy and volume changes associated with the phase transition.
Specifically, it states that dP/dT = ΔH / (TΔV), where dP/dT is the slope of the phase boundary, ΔH is the enthalpy change, T is the temperature, and ΔV is the volume change. The Clausius-Clapeyron equation explains why some phase boundaries have positive slopes (e.g., melting curves of most substances) while others have negative slopes (e.g., the melting curve of water).
Theoretical Modeling of Oxygen Phases
Theoretical modeling provides crucial insights complementing experimental observations. These models, rooted in fundamental physics, allow us to predict and understand the phase behavior of oxygen under diverse conditions.
Quantum Mechanics and Chemical Bonding
Quantum mechanics is essential for understanding the nature of chemical bonds in oxygen. It explains how oxygen atoms interact to form diatomic molecules (O2) and how these molecules interact in the condensed phases (liquid and solid).
The electronic structure of oxygen dictates its bonding characteristics and influences the properties of the various solid phases. For example, different crystal structures arise from variations in the arrangement and interaction of oxygen molecules.
Statistical Mechanics
Statistical mechanics provides a framework for understanding the behavior of macroscopic systems based on the properties of their constituent particles. In the context of oxygen, it helps us to understand how the collective behavior of many oxygen atoms or molecules gives rise to the observed macroscopic properties of each phase.
Statistical mechanics allows us to calculate thermodynamic properties like entropy and free energy, which are crucial for determining phase stability. These calculations often involve complex mathematical techniques and computational simulations.
Density Functional Theory (DFT)
Density Functional Theory (DFT) has become a workhorse for modeling the phase behavior of materials, including oxygen. DFT is a quantum mechanical method that calculates the electronic structure of a system based on its electron density.
DFT simulations can predict the crystal structures, energies, and other properties of different oxygen phases. These predictions can then be used to construct phase diagrams and understand the stability of different phases under varying conditions.
Equations of State
Equations of State (EOS) are mathematical relationships that connect pressure, volume, and temperature for a given substance. They are vital tools for predicting phase equilibria and understanding the behavior of oxygen under extreme conditions. Different EOS models, such as the Van der Waals EOS or more sophisticated models, can be used depending on the desired accuracy and the complexity of the system.
Experimental Techniques for Studying Oxygen Phases
Experimental techniques play a crucial role in validating theoretical models and providing direct observations of oxygen's phase behavior. Several powerful techniques are employed to probe the structure and properties of oxygen under different conditions.
X-ray Diffraction
X-ray diffraction (XRD) is a primary technique for determining the crystal structures of solid phases. By analyzing the diffraction pattern of X-rays scattered by a crystalline material, scientists can determine the arrangement of atoms within the crystal lattice. XRD has been instrumental in elucidating the structures of the various solid oxygen phases.
Neutron Diffraction
Neutron diffraction is complementary to X-ray diffraction. Neutrons interact with the nuclei of atoms rather than the electrons. This makes neutron diffraction particularly useful for studying light elements, such as hydrogen, and for investigating magnetic ordering in materials. It can provide more detailed information about the crystal structure and magnetic properties of oxygen.
Raman Spectroscopy
Raman spectroscopy is a vibrational spectroscopy technique that probes the vibrational modes of molecules and solids. By analyzing the Raman spectrum, scientists can identify different phases and monitor phase transitions. Raman spectroscopy is sensitive to changes in the molecular structure and bonding environment of oxygen.
Percy Williams Bridgman's Contributions
Percy Williams Bridgman was a pioneering physicist who made significant contributions to high-pressure physics. His work on developing high-pressure techniques and studying the properties of materials under extreme conditions laid the foundation for much of the current research on oxygen's phase diagram. While not a specific experimental technique, recognizing Bridgman's contribution is important for historical context in the study of high-pressure phase transitions.
Having armed ourselves with the fundamental principles of phase diagrams, we can now embark on a journey into the intricate world of oxygen's phases. The oxygen phase diagram is not a simple affair; it is a rich tapestry of solid, liquid, and gaseous states, each with its unique characteristics and behaviors under specific conditions. What sets oxygen apart is its stunning variety of solid phases, each emerging under different pressure and temperature regimes. But what happens when we introduce factors beyond simple temperature and pressure variations?
Factors Influencing the Oxygen Phase Diagram: Beyond Basic Conditions
While temperature and pressure are the primary determinants of oxygen's phase, the reality is often more nuanced. The idealized phase diagram, constructed under carefully controlled conditions, represents a pure system. In real-world scenarios, however, the presence of impurities, the introduction of alloying elements, and the application of external fields can significantly alter the phase behavior of oxygen. These factors introduce complexities that must be understood to accurately predict and control oxygen's behavior in various applications.
Temperature and Pressure: The Foundational Parameters
Before exploring the less obvious influences, it's crucial to reiterate the fundamental roles of temperature and pressure. Temperature dictates the kinetic energy of the molecules, influencing their movement and the tendency to transition to higher entropy states like gases. Conversely, pressure favors denser phases by minimizing volume and reducing the overall energy of the system. The interplay between these two parameters defines the basic structure of the oxygen phase diagram, establishing the boundaries between solid, liquid, and gaseous states. Understanding how these parameters interact forms the foundation for appreciating the impact of other factors.
The Role of Impurities and Alloying Elements
The introduction of impurities or alloying elements into an oxygen system can significantly perturb its phase behavior. Impurities, even in small concentrations, can disrupt the crystal lattice structure of solid phases, leading to changes in their stability ranges and transition temperatures.
Impurities
Impurities can act as nucleation sites for new phases or impede phase transitions by pinning phase boundaries. The effect of an impurity depends greatly on its size, charge, and chemical affinity for oxygen. For example, even trace amounts of noble gases can dramatically affect the temperatures and pressures at which oxygen condenses or solidifies.
Alloying Elements
Alloying elements, deliberately introduced to modify oxygen's properties, can have even more profound effects. By forming new chemical bonds with oxygen, alloying elements can stabilize or destabilize specific phases, leading to shifts in phase boundaries and the emergence of entirely new phases.
Consider, for example, the potential effect of introducing nitrogen into solid oxygen. Nitrogen, with its similar size and bonding characteristics, could substitute for oxygen atoms in the lattice structure. Depending on the concentration and temperature, this substitution could either stabilize existing phases, shift phase boundaries, or even induce the formation of entirely novel nitrogen-oxygen compounds. These alloying elements are often used to alter properties and optimize materials for specific applications.
Influence of External Fields: Magnetic and Electric
External fields, such as magnetic and electric fields, can also exert a subtle but measurable influence on the oxygen phase diagram. This influence stems from the interaction of these fields with the electronic structure and magnetic properties of oxygen molecules and solids.
Magnetic Fields
Oxygen, in its molecular form (O2), is paramagnetic due to the presence of unpaired electrons. This paramagnetism means that oxygen molecules are weakly attracted to magnetic fields. Applying a strong magnetic field can therefore influence the alignment of oxygen molecules, particularly in the solid and liquid phases, where intermolecular interactions are more significant.
At extremely high magnetic fields, it is theorized, a direct influence on the energy balance of phases could occur. This could slightly alter the phase boundaries, although the magnitude of the effect is generally small under typical laboratory conditions. Research at extreme conditions has and will be instrumental in mapping the effects of Magnetism in the Oxygen System.
Electric Fields
Electric fields can interact with the electronic structure of oxygen molecules and solids, influencing the strength of chemical bonds and the stability of different phases. While oxygen itself is not strongly polar, the application of a strong electric field can induce a dipole moment in oxygen molecules, leading to electrostatic interactions that affect phase behavior.
The application of strong electric fields may also influence the kinetics of phase transitions, potentially accelerating or decelerating the formation of new phases. However, the experimental verification and detailed understanding of these effects remain areas of active research.
Having armed ourselves with the fundamental principles of phase diagrams, we can now embark on a journey into the intricate world of oxygen's phases. The oxygen phase diagram is not a simple affair; it is a rich tapestry of solid, liquid, and gaseous states, each with its unique characteristics and behaviors under specific conditions. What sets oxygen apart is its stunning variety of solid phases, each emerging under different pressure and temperature regimes. But what happens when we introduce factors beyond simple temperature and pressure variations?
The answer lies in the multifaceted applications where understanding oxygen's phase behavior becomes not just academic, but absolutely critical. From the fiery heart of steelmaking to the delicate balance of life support systems, and even to the crushing depths of planetary interiors, oxygen's phases play a central role.
Applications and Significance: Oxygen's Impact Across Fields
The oxygen phase diagram, seemingly an abstract scientific construct, resonates profoundly across a spectrum of applications. Its influence permeates industrial processes, medical treatments, and cutting-edge scientific inquiries. Understanding oxygen's behavior under various conditions is paramount to optimizing these applications and pushing the boundaries of innovation.
Industrial Applications: The Breath of Modern Manufacturing
Oxygen is a cornerstone of many industrial processes. The steelmaking industry, for example, relies heavily on oxygen to remove impurities from molten iron, enhancing the strength and quality of the final product.
The controlled injection of oxygen into blast furnaces and converters relies on understanding its behavior at high temperatures.
Chemical processes, such as the production of ethylene oxide (a key ingredient in plastics and detergents) and various oxidation reactions, also depend on precise control of oxygen's phase and reactivity. Optimization of these processes hinges on a thorough understanding of oxygen's phase diagram. The efficiency, safety, and yield of these industrial operations are directly linked to the ability to manipulate and predict oxygen's behavior.
Medical Applications: Sustaining Life and Enhancing Treatment
In the realm of medicine, oxygen is literally the breath of life. Oxygen therapy, used to treat respiratory illnesses like pneumonia and chronic obstructive pulmonary disease (COPD), depends on delivering oxygen in its gaseous phase.
Understanding oxygen's solubility in blood and its behavior under different physiological conditions is vital for effective treatment.
Life support systems, used in hospitals, ambulances, and even space exploration, must reliably supply oxygen in a controlled manner. The design and operation of these systems require a firm grasp of oxygen's phase transitions and properties. Furthermore, hyperbaric oxygen therapy, which involves breathing pure oxygen in a pressurized chamber, leverages the principles of gas solubility and diffusion to enhance healing and combat infections.
Scientific Research: Probing the Universe and Unveiling New Frontiers
The study of oxygen phases extends far beyond terrestrial applications. In planetary science, understanding the behavior of oxygen under the extreme pressures and temperatures found in planetary interiors is crucial for modeling the structure and evolution of planets like Earth and Mars.
The discovery of novel solid oxygen phases at ultra-high pressures has provided valuable insights into the fundamental properties of matter and challenged existing theoretical models.
High-pressure physics, which aims to understand the behavior of materials under extreme conditions, heavily relies on studying oxygen and other elements at immense pressures. These studies not only advance our fundamental understanding of matter but also have implications for developing new materials with novel properties.
Importance in Materials Science: A Fundamental Building Block
Oxygen is a ubiquitous element in materials science. It forms oxides with almost every element in the periodic table, creating a vast array of materials with diverse properties. Understanding the phase diagrams of these oxides is critical for designing materials with tailored electrical, magnetic, and mechanical characteristics.
For example, the properties of high-temperature superconductors, many of which are complex oxides, are highly sensitive to oxygen stoichiometry and phase stability.
Controlling the oxygen content and phase distribution in these materials is crucial for optimizing their performance. Furthermore, the study of oxygen diffusion and reactivity is essential for understanding corrosion, oxidation, and other degradation mechanisms that affect the durability of materials in various environments. Oxygen vacancies in materials can also significantly affect conductivity and other important factors.
Future Research Directions: Pushing the Boundaries of Knowledge
While our understanding of oxygen's phases has grown significantly, many tantalizing questions remain, beckoning future research endeavors. The oxygen phase diagram continues to offer fertile ground for exploration, particularly in extreme conditions and through the refinement of theoretical models. Unraveling these remaining mysteries promises to not only deepen our fundamental understanding of matter but also potentially unlock new technological innovations.
The Unexplored Realms of Extreme Conditions
The high-pressure, high-temperature regimes represent a frontier ripe with potential discoveries. Simulating and studying these conditions, often found in planetary interiors or during explosive events, presents formidable experimental and theoretical challenges.
Current research is limited by the capabilities of existing experimental setups and the computational cost of accurate simulations.
However, advancements in diamond anvil cell technology, coupled with high-powered lasers and increasingly sophisticated computational methods, are opening new doors.
These advancements could reveal novel phase transitions and exotic states of oxygen that are currently beyond our grasp. For instance, could oxygen transition to a metallic state at exceedingly high pressures, similar to hydrogen? What role does oxygen play in the chemical reactions occurring in the Earth's mantle or the cores of other planets?
The Quest for Novel Solid Phases
The already impressive array of solid oxygen phases hints at the possibility of even more exotic structures waiting to be discovered. These undiscovered phases might exhibit unique properties such as novel superconductivity or magnetism.
Synthesizing and characterizing these phases require innovative experimental techniques and advanced theoretical predictions. Researchers are exploring the use of novel compression methods, as well as chemical doping and alloying strategies, to stabilize new oxygen phases.
Computational materials science plays a crucial role in predicting the stability and properties of hypothetical phases, guiding experimental efforts. The development of more accurate and efficient computational methods, particularly those that can account for the complex electronic structure of oxygen, is paramount.
Refining Theoretical Models: Bridging Theory and Experiment
Theoretical models are essential tools for understanding and predicting the behavior of oxygen under various conditions. However, current models often struggle to accurately capture the complexities of the oxygen phase diagram, particularly at high pressures and temperatures.
Improving the accuracy of these models requires incorporating advanced quantum mechanical and statistical mechanical techniques.
Density Functional Theory (DFT), a widely used method for electronic structure calculations, is constantly being refined to better account for electron correlation effects, which are particularly important in oxygen.
Moreover, the development of more sophisticated equations of state that can accurately predict phase equilibria is an ongoing area of research. Bridging the gap between theory and experiment requires close collaboration between theoretical and experimental researchers.
By comparing theoretical predictions with experimental observations, researchers can identify the strengths and weaknesses of existing models and develop more accurate and reliable theoretical frameworks. This iterative process is crucial for advancing our understanding of oxygen's phases and predicting its behavior under extreme conditions.
References: A Gateway to Deeper Understanding
The exploration of oxygen's phases, with its surprising complexity and far-reaching implications, draws upon a rich body of scientific literature. This section provides a curated list of references, acting as a launchpad for readers eager to delve deeper into specific aspects of this fascinating topic. These resources span seminal research papers, comprehensive reviews, and insightful books, offering diverse perspectives and methodologies.
A thorough understanding of the oxygen phase diagram necessitates engaging with both theoretical frameworks and experimental findings. The references presented here reflect this duality, encompassing works that explore the fundamental thermodynamic principles governing phase transitions, as well as studies that detail the experimental techniques used to probe the behavior of oxygen under extreme conditions.
Foundational Texts and Landmark Studies
Several key publications have laid the groundwork for our current understanding of oxygen's phases. These foundational texts often provide a broad overview of the field, while landmark studies present groundbreaking experimental or theoretical results that have significantly advanced the field.
Careful examination of these resources is invaluable for any researcher or student seeking a comprehensive grasp of the subject. They represent the cornerstones upon which subsequent research has been built. Expect this section to include core academic texts like "Phase Transitions in Matter" by David A. Young, which is a perfect example for a foundational text.
Cutting-Edge Research and Emerging Trends
Beyond the foundational texts, it is crucial to stay abreast of the latest developments in the field. This includes exploring recent research papers that report novel experimental observations, theoretical models, or computational simulations.
Journals such as Physical Review Letters, Nature, and Science are often at the forefront of these discoveries.
Specifically, research focusing on high-pressure and low-temperature phases is expected to rapidly evolve. This will have a corresponding impact on references that can be provided in this area. Staying current ensures that you're building your knowledge on the best and most recent work.
Resources for Specific Areas of Interest
The study of oxygen's phases encompasses a wide range of sub-disciplines, from high-pressure physics to quantum chemistry.
To facilitate more targeted exploration, the references are categorized by specific areas of interest. This allows readers to quickly identify resources that are relevant to their particular research question or area of study.
Theoretical Modeling and Computation
For those interested in the theoretical underpinnings of oxygen's phase behavior, this section highlights publications that focus on thermodynamic modeling, quantum mechanical calculations, and computational simulations. These resources delve into the equations of state, density functional theory (DFT), and molecular dynamics simulations used to predict and interpret experimental observations.
Experimental Techniques and Data Analysis
This section features references that describe the experimental techniques used to study oxygen's phases, such as X-ray diffraction, neutron scattering, and Raman spectroscopy. It also includes publications that discuss the methods used to analyze experimental data and extract meaningful information about the structure and properties of different phases.
Applications and Technological Implications
Understanding the phase diagram of oxygen has significant implications for a variety of applications, from industrial processes to medical technologies. This section highlights resources that explore the practical applications of oxygen's phase behavior and discuss its role in different technological contexts.
Video: Decoding the Oxygen Phase Diagram: The Ultimate Guide
Oxygen Phase Diagram FAQs
Here are some frequently asked questions about the oxygen phase diagram and understanding its complexities.
What are the stable phases of oxygen under different pressures and temperatures?
The oxygen phase diagram shows that oxygen exists in various solid, liquid, and gaseous phases depending on the pressure and temperature. At low pressures and temperatures, it's a gas. Increasing pressure can solidify oxygen into different crystal structures, each representing a unique phase.
What information can I get from an oxygen phase diagram?
An oxygen phase diagram shows the stable phases of oxygen under different conditions. It tells you what phase of oxygen (solid, liquid, or gas, and the specific solid form) is present at a given temperature and pressure. This is vital in various scientific and industrial applications.
Why does oxygen have so many solid phases?
Oxygen exhibits multiple solid phases due to the different ways oxygen molecules can arrange themselves in a crystal lattice under high pressure. These varying arrangements lead to distinct crystal structures, each representing a stable phase on the oxygen phase diagram.
How is the oxygen phase diagram used in scientific research?
Scientists use the oxygen phase diagram to understand the behavior of oxygen under extreme conditions, such as those found deep within planets or in high-pressure experiments. This understanding helps in fields like materials science, geophysics, and planetary science.