Nano Angstrom: The Science You MUST Know (Explained!)
Nanotechnology, a field reliant on precise measurements, utilizes the nano angstrom as a crucial unit for defining atomic and molecular distances; the scanning tunneling microscope (STM), as developed at IBM Research - Zurich, enables scientists to visualize and manipulate materials at this scale. Precise measurements at the nano angstrom level are critical for advancements in materials science, a domain where properties dramatically change depending on the arrangement of atoms; mastering an understanding of the nano angstrom is therefore fundamental for anyone exploring these groundbreaking fields.
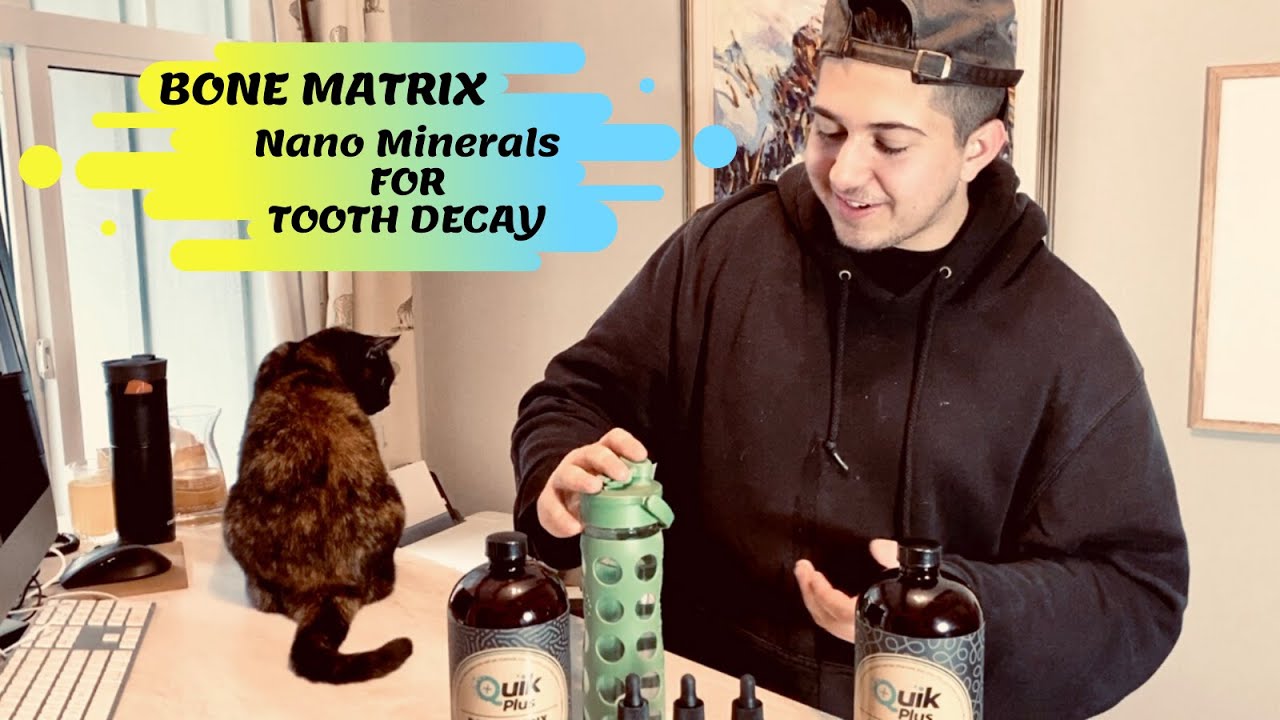
Image taken from the YouTube channel Listen To Your Gut - Jini Patel Thompson , from the video titled How I Use Bone Matrix (nano/angstrom) Atom-Sized Minerals .
In the relentless pursuit of scientific advancement, our ability to probe and manipulate matter at increasingly smaller scales has become paramount. The nanometer and the angstrom—units representing dimensions almost unfathomably tiny—are not merely abstract concepts; they are the keys to unlocking a new era of technological innovation.
Understanding these scales is fundamental for anyone seeking to grasp the inner workings of modern science and technology, and this section serves as your entry point into this fascinating world.
Defining the Infinitesimal: Nano and Angstrom
Let's begin by defining our terms. The prefix "nano" signifies one billionth (10-9) of a meter.
A nanometer (nm) is therefore one billionth of a meter. To put this into perspective, a human hair is roughly 80,000 to 100,000 nanometers wide.
The angstrom (Å) takes us even deeper into the realm of the minuscule. One angstrom is equal to 0.1 nanometers, or 10-10 meters.
This unit is particularly useful when dealing with atomic dimensions, as it closely corresponds to the typical size of an atom.
Why These Scales Matter: Relevance Across Disciplines
The nanometer and angstrom scales are not confined to theoretical physics or advanced chemistry labs. They are central to a vast range of scientific and technological fields, each leveraging the unique properties that emerge at these dimensions.
-
Materials science relies on manipulating materials at the nanoscale to create substances with enhanced strength, conductivity, or reactivity.
-
Medicine is being revolutionized by targeted drug delivery systems and nanoscale diagnostics.
-
Electronics continually pushes the boundaries of miniaturization, developing faster and more efficient transistors with features measured in nanometers.
-
Chemistry benefits by observing molecular interactions and structural determination at angstrom resolution.
A Glimpse into the Applications of Nano Angstrom Science
Nanotechnology and angstrom-level science are not just theoretical pursuits; they have tangible real-world applications that are rapidly transforming various aspects of our lives.
In materials science, for example, researchers are engineering novel nanomaterials like carbon nanotubes and graphene, which exhibit exceptional strength, electrical conductivity, and thermal properties. These materials are finding their way into everything from lightweight composites for aerospace to high-performance batteries for electric vehicles.
Medicine is also undergoing a revolution thanks to nanotechnology. Nanoparticles are being developed to deliver drugs directly to cancer cells, minimizing side effects and maximizing therapeutic efficacy. Similarly, nanoscale sensors are enabling earlier and more accurate diagnoses of diseases.
What to Expect in This Exploration
This blog post will delve into the depths of the nano and angstrom scales, exploring the tools, techniques, and applications that make this realm so compelling. We will be covering:
-
An expanded explanation of the nanoscale and its unique characteristics.
-
A detailed look at the angstrom unit and its significance in measuring atomic dimensions.
-
An overview of the advanced tools used to visualize and manipulate matter at these scales, such as Scanning Tunneling Microscopy (STM) and Atomic Force Microscopy (AFM).
-
A showcase of real-world applications of nanotechnology and angstrom-level science across diverse fields.
-
A glimpse into the future, highlighting emerging trends and potential breakthroughs in this rapidly evolving area.
Materials science relies on manipulating materials at the nanoscale to create substances with enhanced strength, conductivity, or reactivity. Medicine is being revolutionized by targeted drug delivery systems and nanoscale diagnostics. Electronics continually pushes the boundaries of miniaturization, developing components so small they operate on principles governed by quantum mechanics. To truly grasp the scope of these advancements, we need to explore the defining characteristics of the nanoscale itself.
The Nanoscale Explained: Defining the Realm of the Very Small
The nanoscale is generally defined as the range from 1 to 100 nanometers. This seemingly arbitrary boundary marks a critical transition where the properties of matter often deviate significantly from those observed at larger, macroscopic scales.
Defining the Boundaries
While the nanoscale is commonly accepted to span 1-100 nm, it's important to understand why this particular range is so significant.
Below 1 nm, we are essentially dealing with individual atoms and small molecules, where the properties are primarily dictated by quantum mechanics. Above 100 nm, materials typically begin to exhibit bulk-like behavior, where classical physics provides a more accurate description.
Therefore, the 1-100 nm range represents a fascinating intermediate regime where both classical and quantum effects play a crucial role, leading to novel and often unexpected phenomena.
Emergent Properties at the Nanoscale
Perhaps the most compelling aspect of the nanoscale is the emergence of size-dependent properties. These are characteristics that are not simply scaled-down versions of their macroscopic counterparts.
One of the most significant factors driving these changes is the dramatic increase in surface area to volume ratio.
As a particle's size decreases, the proportion of atoms residing on its surface increases exponentially. This has profound implications for properties like:
-
Reactivity: Nanomaterials often exhibit dramatically enhanced reactivity due to the increased availability of surface atoms for chemical reactions.
-
Mechanical Strength: The strength and elasticity of materials can be significantly altered at the nanoscale, leading to the development of ultra-strong composites and flexible electronics.
-
Optical Properties: The way nanomaterials interact with light can be drastically different from their bulk counterparts, leading to applications in areas like sensing, imaging, and photovoltaics.
Implications Across Disciplines
The unique properties exhibited by materials at the nanoscale have opened up a vast array of possibilities across various scientific and technological fields.
Materials Science
In materials science, researchers are leveraging nanoscale control to design materials with unprecedented properties. For example, carbon nanotubes, with diameters on the order of a few nanometers, possess exceptional strength and electrical conductivity, making them ideal for applications ranging from lightweight composites to high-performance electronics.
Medicine
In the realm of medicine, nanoparticles are being explored for targeted drug delivery. These tiny carriers can be engineered to selectively target cancer cells or other diseased tissues, minimizing side effects and maximizing therapeutic efficacy.
Nanoscale diagnostics are also emerging, enabling the early detection of diseases through the detection of subtle biomarkers at the molecular level.
A Glimpse into Quantum Mechanics
At the nanoscale, the principles of classical physics begin to break down, and the effects of quantum mechanics become increasingly prominent. This is because the de Broglie wavelength of electrons, which dictates their wave-like behavior, becomes comparable to the size of the nanostructures.
As a result, phenomena such as quantum tunneling and quantum confinement can significantly influence the behavior of electrons in nanomaterials, leading to unique electronic and optical properties.
Understanding these quantum effects is crucial for designing and developing advanced nanoscale devices, such as quantum dots and single-electron transistors, which hold immense promise for future technological innovations.
The exploration of materials and medicine at the nanoscale naturally leads us to consider the even finer resolution offered by the angstrom unit. While the nanometer provides a useful scale for understanding many structural phenomena, the angstrom allows us to delve into the realm of individual atoms and the bonds that hold them together. This unit of measurement is not just a smaller increment; it is the key to unlocking our understanding of the atomic architecture of matter.
Angstrom: The Atomic Unit of Measurement
The angstrom (Å) is a unit of length equal to 0.1 nanometers (nm), 10-10 meters, or 100 picometers (pm).
It is not an SI unit but is still widely used in fields like chemistry, solid-state physics, and structural biology.
Angstrom and Atomic Dimensions
The angstrom is particularly useful because it approximates the typical size of an atom and the length of chemical bonds.
For example, the radius of a hydrogen atom is about 0.5 Å, and the length of a typical carbon-carbon single bond is around 1.5 Å.
This close correspondence makes the angstrom an intuitive and convenient unit for describing atomic and molecular structures.
Relating Angstroms to Other Units
To put the angstrom into perspective, it's helpful to compare it to other units of measurement commonly used in science and technology.
- Meter (m): The base unit of length in the SI system. One angstrom is one ten-billionth of a meter (1 Å = 10-10 m).
- Nanometer (nm): One nanometer is equal to 10 angstroms (1 nm = 10 Å). It's commonly used to describe the size of nanoparticles and features in microelectronics.
- Picometer (pm): One picometer is one-tenth of an angstrom (1 pm = 0.01 Å). It's often used in high-resolution structural studies.
Visualizing the Angstrom Scale
It can be challenging to grasp the scale of an angstrom without visual aids.
Imagine a row of ten hydrogen atoms lined up side by side; their total length would be approximately 1 nanometer, with each atom spanning about 1 angstrom across.
Similarly, consider a water molecule (H2O); the distance between the oxygen atom and each hydrogen atom is roughly 1 angstrom.
Visualizing molecules and materials at this scale helps to appreciate the precision required to manipulate matter at the atomic level. Tools like X-ray crystallography provide detailed structural information with angstrom-level resolution, revealing the intricate arrangements of atoms in crystals and molecules. These techniques are crucial for understanding the properties and functions of materials, from pharmaceuticals to semiconductors.
The ability to perceive and interact with matter at the nano and angstrom scales represents a pivotal advancement in modern science. Yet, even with a solid understanding of what these minute measurements entail, it begs the question: how exactly do scientists see and manipulate objects so incredibly small?
Key Tools and Techniques for Observing the Nano Angstrom
The exploration of the nano and angstrom realms relies on sophisticated instrumentation capable of resolving individual atoms and molecules. These tools, operating on principles of quantum mechanics and advanced optics, allow researchers to not only visualize but also manipulate matter with unprecedented precision. Let's delve into some of the most prominent techniques:
Scanning Tunneling Microscopy (STM)
Scanning Tunneling Microscopy (STM) is a technique renowned for its ability to achieve true atomic resolution imaging of conductive surfaces.
Principles of STM
STM operates on the principle of quantum tunneling. A sharp, conductive tip is brought extremely close (within a few angstroms) to the surface of a sample.
A voltage is applied between the tip and the sample, and due to their close proximity, electrons can "tunnel" through the potential energy barrier between them, even though classically they should not have enough energy to overcome it.
This tunneling current is exquisitely sensitive to the distance between the tip and the surface. By maintaining a constant tunneling current, a feedback loop adjusts the tip's vertical position as it scans across the surface, effectively mapping the surface topography at the atomic level.
Atomic Resolution Imaging with STM
The exponential dependence of the tunneling current on the tip-sample distance allows STM to resolve features as small as 0.1 angstroms laterally and 0.01 angstroms vertically.
This capability makes STM indispensable for studying surface structures, identifying adsorbed atoms, and even manipulating individual atoms on a surface.
Atomic Force Microscopy (AFM)
Atomic Force Microscopy (AFM) expands upon the principles of STM by enabling the imaging of both conductive and non-conductive surfaces.
Principles of AFM
AFM utilizes a sharp tip attached to a cantilever, a small beam that vibrates at a specific frequency. As the tip scans across the sample surface, it experiences forces (both attractive and repulsive) from the atoms on the surface.
These forces cause the cantilever to bend or deflect. A sensor measures this deflection, allowing the instrument to create an image of the surface topography.
Modes of Operation: Contact and Non-Contact
AFM offers various modes of operation, each suited for different types of samples and imaging requirements.
In contact mode, the tip is in direct contact with the surface, and the cantilever deflection is maintained at a constant level.
Non-contact mode involves oscillating the cantilever near its resonant frequency and sensing changes in the oscillation amplitude or frequency due to surface forces. This mode is gentler than contact mode and is often preferred for delicate samples.
There's also Tapping Mode where the cantilever oscillates near its resonant frequency but taps the surface lightly during scanning. This minimizes lateral forces and is often used for imaging soft materials like biological samples.
Electron Microscopy: TEM and SEM
Electron microscopy provides high-resolution imaging by utilizing beams of electrons instead of light. Because electrons have much smaller wavelengths than visible light, they can achieve significantly higher magnifications and resolutions.
Transmission Electron Microscopy (TEM) vs. Scanning Electron Microscopy (SEM)
Transmission Electron Microscopy (TEM) involves transmitting a beam of electrons through an ultra-thin sample. The electrons interact with the sample, and the transmitted electrons are used to create an image. TEM provides information about the internal structure of the sample at the nanometer and angstrom scales.
Scanning Electron Microscopy (SEM), on the other hand, scans the surface of a sample with a focused electron beam. The electrons interact with the sample, producing various signals (e.g., secondary electrons, backscattered electrons) that are detected to create an image of the surface topography.
Applications in Materials Science and Biology
TEM is widely used in materials science to study the microstructure of materials, including crystal defects, grain boundaries, and phase distributions. In biology, TEM is used to image cells, viruses, and other biological structures at high resolution.
SEM is commonly used to study the surface morphology of materials, analyze fractures, and examine the structure of biological specimens. It often requires coating the sample with a thin conductive layer (e.g., gold) to improve image quality.
X-ray Diffraction (XRD)
While not a direct imaging technique, X-ray Diffraction (XRD) is an indispensable tool for determining the atomic structure of crystalline materials.
XRD involves directing a beam of X-rays onto a crystalline sample. The X-rays are diffracted by the atoms in the crystal lattice, and the resulting diffraction pattern is analyzed to determine the arrangement of atoms and the spacing between them.
XRD is crucial for identifying crystal structures, determining lattice parameters, and analyzing the composition of materials. It provides valuable information about the atomic-level structure of solids.
Applications of Nano Angstrom Science: Where We Use This Knowledge
The ability to observe and manipulate matter at the nano and angstrom scales represents a pivotal advancement in modern science. Yet, even with a solid understanding of what these minute measurements entail, it begs the question: how exactly do scientists see and manipulate objects so incredibly small?
As our ability to observe and manipulate materials at the nano and angstrom scales has progressed, so too have the applications of this knowledge. From revolutionizing materials science to transforming medicine and electronics, the impact of nanotechnology is already being felt across numerous sectors, offering solutions to previously insurmountable challenges.
Materials Science: Engineering the Future Atom by Atom
Nanomaterials, designed and engineered at the atomic level, exhibit remarkable properties that surpass those of their bulk counterparts. This opens the door to creating materials with unprecedented strength, enhanced conductivity, and tailored functionalities, driving innovation across various industries.
Carbon Nanotubes: Strength and Versatility
Carbon nanotubes (CNTs), cylindrical molecules composed of rolled-up sheets of graphene, are a prime example of the power of nanomaterials. Their exceptional tensile strength, often exceeding that of steel, combined with their remarkable electrical and thermal conductivity, makes them ideal for applications ranging from lightweight composites in aerospace to high-performance electronics.
CNTs are finding use in advanced sporting equipment, reinforcing polymers, and even in developing next-generation sensors. Their ability to act as both structural components and functional elements underscores their versatility and potential for widespread adoption.
Graphene: A Revolutionary 2D Material
Graphene, a single-layer sheet of carbon atoms arranged in a hexagonal lattice, is another nanomaterial that has captured the imagination of scientists and engineers. Its exceptional strength, flexibility, and electrical conductivity make it a promising candidate for a wide range of applications.
Graphene is being explored for use in flexible electronics, high-performance batteries, and advanced filtration systems. Its large surface area and unique electronic properties also make it attractive for use in sensors and catalysts. Research continues to unlock new potential uses for this revolutionary 2D material.
Medicine: Revolutionizing Healthcare at the Molecular Level
Nanotechnology is transforming the landscape of medicine, offering innovative approaches to drug delivery, diagnostics, and therapies. By manipulating materials at the nanoscale, researchers are developing targeted drug delivery systems, highly sensitive diagnostic tools, and novel therapeutic interventions.
Nanoparticles for Targeted Drug Delivery
Traditional drug delivery methods often result in systemic exposure, leading to unwanted side effects and reduced efficacy. Nanoparticles offer a solution by encapsulating drugs and delivering them directly to the targeted cells or tissues, minimizing off-target effects and maximizing therapeutic impact.
These nanoparticles can be engineered to respond to specific stimuli, such as pH or temperature, releasing their payload only at the site of the disease. This targeted approach holds immense promise for treating cancer, infectious diseases, and other conditions with greater precision and fewer side effects.
Nanoscale Diagnostics: Early Detection and Personalized Medicine
Nanoscale diagnostic tools are enabling earlier and more accurate detection of diseases, paving the way for personalized medicine approaches. These tools leverage the unique properties of nanomaterials to detect biomarkers, image tissues, and monitor physiological processes with unprecedented sensitivity.
For example, quantum dots, fluorescent semiconductor nanocrystals, can be used to label and track cells, allowing researchers to visualize biological processes in real time. Nanobiosensors, incorporating nanomaterials, can detect even trace amounts of disease biomarkers in blood or urine, enabling early diagnosis and personalized treatment strategies.
Electronics: Pushing the Boundaries of Computing and Energy
Nanotechnology is playing a crucial role in the ongoing revolution in electronics, enabling the development of smaller, faster, and more energy-efficient devices. By manipulating materials at the nanoscale, researchers are pushing the boundaries of computing, energy storage, and solar energy conversion.
Smaller and Faster Transistors: Moore's Law Continues
The relentless drive to shrink transistors, the fundamental building blocks of computers, has been a major force behind the exponential growth in computing power known as Moore's Law. Nanotechnology is enabling the creation of transistors with features sizes of just a few nanometers, packing more transistors onto a single chip and increasing processing speeds.
Nanomaterials, such as carbon nanotubes and graphene, are being explored as replacements for silicon in transistors, potentially leading to even smaller and faster devices. These advancements will continue to drive innovation in computing, enabling new applications in artificial intelligence, data analytics, and other fields.
Nanomaterials for Solar Cells and Batteries: Sustainable Energy Solutions
Nanotechnology is also contributing to the development of more efficient and sustainable energy technologies. Nanomaterials are being used to enhance the performance of solar cells, increasing their efficiency and reducing their cost. They are also being used to create batteries with higher energy density, faster charging times, and longer lifespans.
For example, nanoparticles can be incorporated into solar cells to improve light absorption and energy conversion. Nanostructured electrodes in batteries can increase the surface area available for electrochemical reactions, leading to higher energy storage capacity. These advancements are critical for transitioning to a cleaner and more sustainable energy future.
The Future of Nano Angstrom: Emerging Trends and Possibilities
As we delve deeper into the realms of nanotechnology and angstrom-level science, it's crucial to consider the exciting possibilities that lie ahead. The current advancements hint at a future where materials are engineered with unprecedented precision, medical treatments are personalized at the molecular level, and energy solutions are more efficient and sustainable. This section will explore these emerging trends, emphasizing both the potential benefits and the ethical considerations that must guide our exploration.
Revolutionizing Energy Through Nanotechnology
The energy sector stands to gain significantly from advancements in nano angstrom science. Nanomaterials are being developed to enhance the efficiency of solar cells, allowing for greater energy conversion from sunlight. Quantum dots, for example, are being explored for their ability to absorb a broader spectrum of light compared to traditional silicon-based solar cells.
Furthermore, nanotechnology plays a critical role in developing advanced batteries with higher energy density and faster charging capabilities. Nanostructured electrodes and electrolytes are being designed to improve ion transport and reduce internal resistance, leading to more efficient and durable batteries.
This is particularly relevant for electric vehicles and grid-scale energy storage.
Personalized Medicine at the Molecular Level
Nanotechnology is poised to transform medicine by enabling personalized treatments tailored to individual patients. Nanoparticles can be engineered to deliver drugs directly to cancer cells, minimizing side effects and improving therapeutic outcomes.
These targeted drug delivery systems can be designed to respond to specific biomarkers or stimuli within the body, releasing their payload only when and where it is needed. Nanoscale diagnostic tools are also emerging, capable of detecting diseases at their earliest stages with unparalleled sensitivity.
These tools can analyze individual molecules or cells, providing clinicians with detailed information about a patient's health status.
Nanobots and the Future of Surgery
Imagine microscopic robots, nanobots, navigating through the human body to repair damaged tissues or perform complex surgical procedures. While still in its early stages, research in this area is progressing rapidly.
These nanobots could be programmed to target specific cells or structures, delivering drugs, removing clots, or even performing microsurgery with extreme precision.
Advanced Materials: Engineering the Impossible
The development of advanced materials with tailored properties is another exciting frontier in nano angstrom science. Researchers are creating materials with unprecedented strength, flexibility, and conductivity by manipulating their atomic structure.
Graphene, with its exceptional strength and conductivity, is just one example of the potential of these materials. Other nanomaterials, such as carbon nanotubes and nanowires, are being explored for applications in aerospace, construction, and electronics.
These materials could enable the creation of lighter, stronger, and more durable structures, as well as more efficient electronic devices.
Quantum Computing: Harnessing the Power of the Quantum World
Quantum computing promises to revolutionize computation by harnessing the principles of quantum mechanics. At the heart of quantum computing are qubits, which can exist in multiple states simultaneously, allowing for exponentially faster computations compared to classical bits.
Nanotechnology plays a crucial role in building and controlling qubits, as these quantum systems are extremely sensitive to their environment. Researchers are exploring various nanomaterials and nanostructures to create stable and scalable qubits.
If successful, quantum computers could solve complex problems that are currently intractable, such as drug discovery, materials design, and financial modeling.
Ethical and Societal Implications
As nanotechnology advances, it is crucial to address the ethical and societal implications of these technologies. Concerns have been raised about the potential toxicity of nanomaterials, the environmental impact of their production and disposal, and the potential for misuse of these technologies.
It is essential to develop regulations and guidelines to ensure that nanotechnology is developed and used responsibly, prioritizing human health and environmental sustainability. Public engagement and education are also crucial to foster informed discussions about the benefits and risks of nanotechnology.
Ultimately, the future of nano angstrom science depends on our ability to harness its potential for good, while carefully considering and mitigating its potential risks.
Video: Nano Angstrom: The Science You MUST Know (Explained!)
Nano Angstrom Science: Frequently Asked Questions
Here are some common questions about the world of nano angstrom measurements and their significance in science and technology.
What exactly is a nano angstrom?
A nano angstrom isn't a standard unit. It's a combination of "nano" (10⁻⁹) and "angstrom" (10⁻¹⁰ meters). Therefore, a nano angstrom would theoretically be 10⁻¹⁹ meters. This is incredibly small – far smaller than even an atom! While not a standard unit, understanding the scales involved helps grasp the minuteness of things at the atomic and subatomic level.
Why is understanding these tiny measurements important?
Understanding the scale of nano angstrom and related measurements is vital in fields like nanotechnology, materials science, and biology. These disciplines often deal with structures and phenomena at the atomic and molecular level.
Precisely controlling and manipulating matter at this scale allows for the creation of new materials with novel properties and functionalities.
How does nanotechnology use measurements related to nano angstrom dimensions?
Nanotechnology relies heavily on precise control at the nano scale and even finer resolutions. Scientists use sophisticated instruments like atomic force microscopes to "see" and manipulate structures.
This allows them to build nanoscale devices and materials with functionalities determined by arrangements measured in angstroms and even perceived in terms related to nano angstrom estimations.
What are some real-world applications of science that deal with these tiny measurements?
Several applications benefit from these measurements. For instance, advanced semiconductors require precise placement of atoms to function correctly. Drug delivery systems can be engineered to target specific cells based on size and molecular interactions.
In materials science, understanding the atomic structure of materials allows scientists to engineer materials with increased strength, conductivity, or other desirable properties. All of this is at least perceived in terms of nano angstrom understanding.
So, that's the skinny on nano angstrom! Hope it all clicked. Now you've got a better handle on this tiny, but mighty, concept.