Measuring Photosynthesis: Ultimate Guide!
Understanding the intricate process of measuring photosynthesis is crucial for various fields, from agricultural optimization to environmental monitoring. Chlorophyll fluorescence, a key technique for assessing plant health, provides valuable data when measuring photosynthesis. Scientists and researchers at institutions like the Carnegie Institution for Science are actively involved in advancing methodologies for measuring photosynthesis. And, tools like the LI-COR portable photosynthesis system offer precise means of measuring photosynthesis in the field, enabling researchers to better understand plant function.
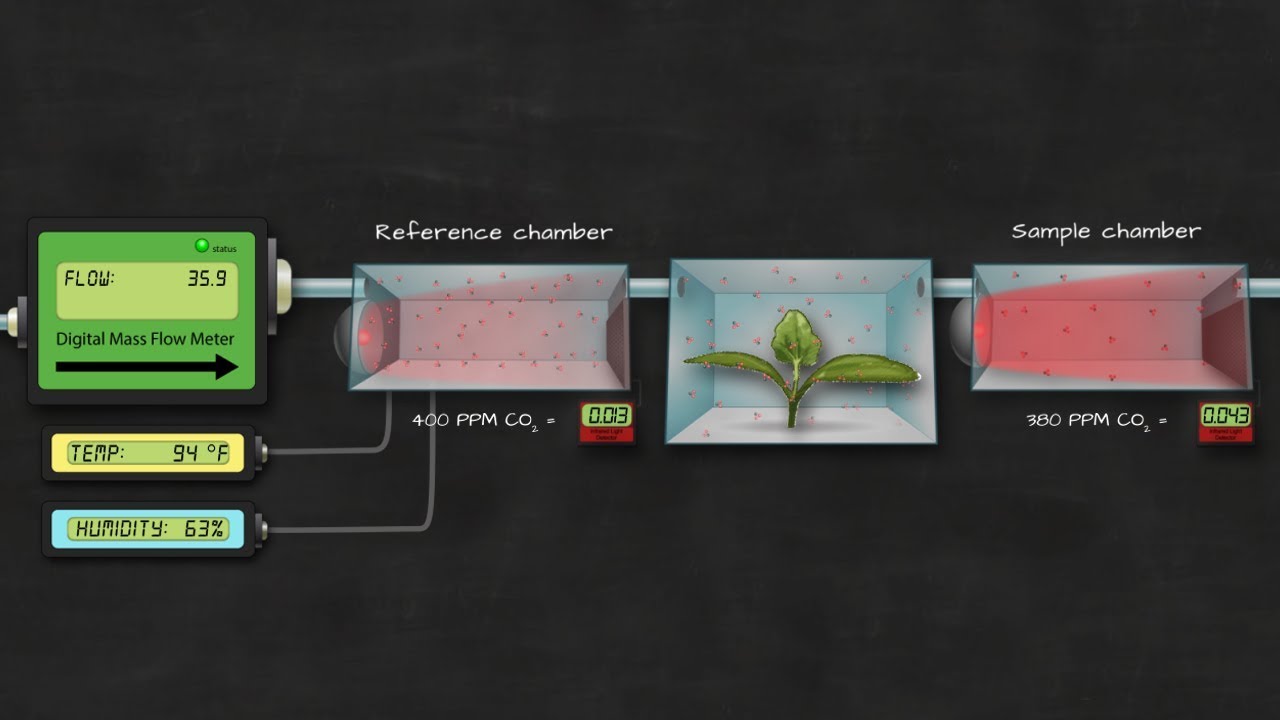
Image taken from the YouTube channel NEON Science , from the video titled How We Measure Photosynthesis .
Photosynthesis, the remarkable process by which plants, algae, and certain bacteria convert light energy into chemical energy, forms the bedrock of nearly all life on Earth. It's the engine that drives our ecosystems, providing the oxygen we breathe and the food we eat. Understanding this fundamental process is not merely an academic exercise; it's a critical endeavor with profound implications for a range of scientific disciplines and real-world applications.
Photosynthesis sustains life by capturing the sun's radiant energy and transforming it into sugars (glucose), which serve as the primary fuel for plant growth and development. As a byproduct, oxygen is released into the atmosphere, making it breathable for animals and humans alike. Without photosynthesis, our planet would be a drastically different, and far less hospitable, place.
Why Measure Photosynthesis? The Broader Significance
The study of photosynthesis transcends the boundaries of basic biology. Accurate measurement and analysis of photosynthetic activity are essential for addressing some of the most pressing challenges facing humanity today.
-
Agriculture: In agriculture, understanding photosynthesis is crucial for optimizing crop yields and developing more efficient farming practices. By monitoring photosynthetic rates, we can identify factors that limit plant growth and implement strategies to enhance productivity, such as optimizing irrigation, fertilization, and light exposure.
-
Environmental Science: Environmental scientists rely on photosynthesis measurements to assess the health of ecosystems and understand the impact of pollution, climate change, and other environmental stressors on plant life. Declining photosynthetic rates can serve as an early warning sign of ecosystem degradation.
-
Climate Change Research: Photosynthesis plays a pivotal role in the global carbon cycle, acting as a major sink for atmospheric carbon dioxide. Measuring photosynthetic rates in various ecosystems helps us understand how effectively these systems are removing carbon dioxide from the atmosphere and mitigating climate change. Accurately measuring and modeling photosynthesis is vital for predicting future climate scenarios and developing effective climate mitigation strategies.
A Guide to Measuring Photosynthesis: What Lies Ahead
This guide provides a comprehensive overview of the methods, factors, and applications involved in measuring photosynthesis. We will delve into the various techniques used to quantify photosynthetic activity, explore the environmental and plant-specific factors that influence these measurements, and showcase the diverse applications of photosynthesis measurement across different fields.
We'll begin by laying out the basics of photosynthesis itself, so we can better understand the process. Then, we will walk through the tools of the trade, including the following:
- Gas Exchange Methods: Measuring carbon dioxide uptake and oxygen release.
- Chlorophyll Fluorescence: Probing the efficiency of photosystems.
- Spectrophotometry: Quantifying pigment concentrations.
We will also explore the factors influencing photosynthesis, including light intensity, carbon dioxide concentration, temperature, and water availability, as well as plant-specific attributes such as leaf age, nutrient status, and stomatal conductance.
Finally, we will explore the applications of this knowledge. By understanding the principles and practices of photosynthesis measurement, we can unlock new insights into the intricate workings of our planet and pave the way for a more sustainable future.
Photosynthesis 101: The Basic Science
Photosynthesis, at its heart, is a remarkably elegant process. Before diving into the intricacies of measuring it, it's crucial to grasp the fundamental science that underpins it all. Think of it as understanding the recipe before you start cooking – knowing the ingredients and their roles is essential for success.
The goal here is to break down the complex mechanisms of photosynthesis into easily digestible pieces, paving the way for a deeper understanding of the measurement techniques discussed later.
The Core Equation: Capturing Sunlight's Energy
The essence of photosynthesis can be captured in a single, deceptively simple equation:
Carbon Dioxide + Water + Light Energy → Glucose + Oxygen
This equation tells a powerful story: plants (and other photosynthetic organisms) take in carbon dioxide from the atmosphere and water from the soil. Using the energy from sunlight, they transform these simple ingredients into glucose (a sugar), which fuels their growth and activities. As a byproduct, they release oxygen back into the atmosphere, the very air we breathe.
It’s more than just a chemical reaction; it's a life-sustaining cycle that underpins virtually all ecosystems on Earth.
Key Players in the Photosynthetic Process
Understanding the equation is a good start, but let's delve deeper into the roles of the key components that make this process possible. Each plays a vital and specific role in the overall functioning of photosynthesis.
Chlorophyll: The Master of Light Absorption
Chlorophyll is the green pigment found in plants (and algae and cyanobacteria). It's the primary light-absorbing molecule, acting like an antenna to capture photons of light. Different types of chlorophyll exist, each absorbing light most efficiently at slightly different wavelengths.
This absorbed light energy is then converted into chemical energy, initiating the cascade of reactions that ultimately lead to glucose production. Without chlorophyll, the light energy needed to drive photosynthesis would simply pass through the plant.
Light Intensity: The Engine's Fuel
Light intensity directly influences the rate of photosynthesis. More light generally means a faster rate, up to a certain point. Plants have a "light saturation point," beyond which increasing light intensity no longer increases photosynthesis, and can even cause damage.
Understanding the light response curve of a plant – how its photosynthetic rate changes with varying light intensities – is crucial in both research and agricultural settings.
Carbon Dioxide: The Building Block of Sugar
Carbon dioxide (CO2) serves as the primary carbon source for building glucose molecules. Plants obtain CO2 from the atmosphere through tiny pores on their leaves called stomata.
The concentration of CO2 around the plant directly impacts the photosynthetic rate. In many environments, CO2 is a limiting factor, meaning that increasing CO2 levels can boost photosynthesis and growth.
Oxygen: The Life-Giving Byproduct
Oxygen (O2) is produced as a byproduct of the water-splitting reaction in photosynthesis. While oxygen is essential for animal life, it's essentially a waste product for the plant itself.
However, the release of oxygen into the atmosphere is what makes our planet habitable.
Photosynthetic Rate: A Measure of Productivity
The photosynthetic rate is a measure of how efficiently a plant is converting light energy into chemical energy. It essentially quantifies the amount of carbon dioxide being fixed (converted into glucose) per unit of time and leaf area.
This rate is a crucial indicator of a plant's health and productivity. Factors like nutrient availability, water stress, and environmental conditions can all significantly influence the photosynthetic rate.
Measuring photosynthetic rate allows scientists and farmers to assess plant performance, identify limiting factors, and optimize growing conditions for maximum yield. It's a powerful tool for understanding how plants respond to their environment and for predicting their growth potential.
Tools of the Trade: Methods for Measuring Photosynthesis
Having explored the fundamental science underpinning photosynthesis, the next crucial step is understanding how we actually measure this vital process. A suite of techniques has been developed, each offering unique insights into different facets of photosynthetic activity. These tools allow scientists to quantify the rate at which plants convert light energy into chemical energy, providing valuable data for research and applications across various disciplines.
Gas Exchange Methods: Measuring CO2 Uptake and O2 Release
One of the most direct ways to measure photosynthesis is by monitoring the exchange of gases involved in the process. Plants consume carbon dioxide (CO2) from the atmosphere and release oxygen (O2) as a byproduct.
By quantifying the rate of CO2 uptake and O2 release, we can directly assess the rate of photosynthesis.
Infrared Gas Analyzers (IRGA): The Workhorse of Gas Exchange
Infrared Gas Analyzers (IRGA) are the primary instruments used in gas exchange measurements.
These devices work on the principle that different gases absorb infrared radiation at specific wavelengths. An IRGA shines a beam of infrared light through a sample of air, and measures the amount of light absorbed by CO2 and water vapor.
By comparing the amount of light absorbed by the sample to a reference, the concentration of the gas can be accurately determined.
Sophisticated systems can precisely control the conditions within a leaf chamber, manipulating factors like light intensity, CO2 concentration, temperature, and humidity.
This allows researchers to investigate how these environmental parameters influence photosynthetic rates.
Applications of IRGA: Unveiling Photosynthetic Responses
IRGA measurements are invaluable for determining photosynthetic rates under a wide range of conditions.
For instance, researchers can use IRGA to construct light response curves, which illustrate how photosynthetic rate changes with varying light intensities.
Similarly, they can investigate the impact of CO2 concentration, temperature, and water stress on photosynthetic performance. These types of measurements can reveal the optimal conditions for photosynthesis in different plant species.
IRGA can also be used to assess the impact of pollution on plant health, or to compare the photosynthetic efficiencies of different plant varieties.
Pros and Cons of Gas Exchange Methods
Gas exchange methods offer several advantages. They provide a direct measure of photosynthetic activity, are relatively simple to operate, and can be used in both laboratory and field settings.
However, they also have some limitations. Gas exchange measurements can be affected by factors such as leaf boundary layer resistance, which can impede gas diffusion.
Furthermore, the equipment can be expensive and require regular calibration. Finally, gas exchange measurements provide a net photosynthetic rate.
That is, the difference between photosynthesis and respiration, meaning that dark respiration must be independently measured and accounted for.
Chlorophyll Fluorescence: Probing the Efficiency of Photosystems
Chlorophyll fluorescence provides a non-destructive way to assess the health and efficiency of photosystems, the protein complexes within chloroplasts that capture light energy.
When chlorophyll molecules absorb light energy, some of that energy is used to drive photosynthesis, some is dissipated as heat, and some is re-emitted as fluorescence.
By measuring the intensity and characteristics of the fluorescence signal, we can gain insights into the efficiency of the photosynthetic process.
Pulse Amplitude Modulation (PAM) Fluorometry: A Powerful Tool
Pulse Amplitude Modulation (PAM) fluorometry is the most widely used technique for measuring chlorophyll fluorescence.
PAM fluorometers emit short pulses of light and measure the resulting fluorescence signal. By analyzing the kinetics of the fluorescence signal, researchers can determine various parameters related to photosynthetic efficiency.
Quantum Yield and Photosystem Health
PAM fluorometry allows the determination of quantum yield, a measure of the efficiency with which light energy is converted into chemical energy.
A high quantum yield indicates that the photosystems are functioning efficiently, while a low quantum yield suggests that the photosystems are stressed or damaged.
PAM fluorometry can also be used to assess the health of photosystems by measuring parameters such as the maximum quantum yield of photosystem II (PSII) (Fv/Fm).
A decrease in Fv/Fm can indicate photoinhibition, a phenomenon where excessive light exposure damages the photosystems.
Advantages of Chlorophyll Fluorescence
Chlorophyll fluorescence offers several key advantages. It is a non-destructive technique, meaning that measurements can be made repeatedly on the same plant without causing damage.
It is also a rapid and sensitive technique, allowing for high-throughput screening of plant populations.
Furthermore, chlorophyll fluorescence provides information about the internal workings of the photosynthetic machinery, offering insights that cannot be obtained from gas exchange measurements alone.
Spectrophotometry: Quantifying Pigment Concentrations
Spectrophotometry is a technique used to measure the absorbance and transmittance of light through a solution. It is based on the principle that different substances absorb light at specific wavelengths.
In the context of photosynthesis, spectrophotometry is used to measure the concentrations of chlorophyll and other photosynthetic pigments in plant tissues.
Measuring Chlorophyll Concentrations
To measure chlorophyll concentration, a sample of leaf tissue is typically ground up in a solvent, such as acetone or ethanol, to extract the pigments.
The extract is then placed in a spectrophotometer, which measures the absorbance of light at specific wavelengths.
The absorbance values are then used to calculate the concentrations of chlorophyll a, chlorophyll b, and other pigments using established equations.
Pigment Levels and Photosynthetic Capacity
The concentration of photosynthetic pigments, particularly chlorophyll, is closely related to photosynthetic capacity.
Plants with higher chlorophyll concentrations generally have higher photosynthetic rates. However, the relationship between pigment levels and photosynthetic capacity is not always straightforward.
Other factors, such as the efficiency of the photosystems and the availability of CO2, can also influence photosynthetic rates.
Spectrophotometry is a valuable tool for assessing plant health and nutritional status, as pigment concentrations can be affected by nutrient deficiencies and other environmental stressors.
Other Measurement Approaches
While gas exchange, chlorophyll fluorescence, and spectrophotometry are the most commonly used techniques for measuring photosynthesis, other approaches can provide valuable complementary information.
For example, Leaf Area Index (LAI), which measures the total leaf area per unit ground area, is often used in ecosystem studies to estimate the overall photosynthetic capacity of a plant canopy.
Measurements of above ground biomass are useful for measuring productivity in long term studies.
Measurements of root carbohydrates are also used in some studies to determine the effects of different environmental conditions on productivity and carbon partitioning in plants.
These include liquid chromatography-mass spectrometry (LC-MS) and high-performance liquid chromatography (HPLC) techniques.
By combining different measurement techniques, researchers can obtain a more comprehensive understanding of the photosynthetic process and its response to various environmental factors.
Under the Microscope: Factors Influencing Photosynthetic Measurements
Having the right tools is only half the battle. Accurately measuring photosynthesis requires a deep understanding of the many factors that can influence the process. Both environmental conditions and the plant's own internal state can dramatically affect photosynthetic rates, making careful consideration of these variables essential for valid data interpretation.
Environmental Factors: Setting the Stage for Photosynthesis
The environment provides the raw materials and energy that drive photosynthesis. Light, carbon dioxide, temperature, and water availability each play a critical role, and variations in these factors can significantly impact photosynthetic activity.
Light Intensity: Riding the Light Response Curve
Light is the primary energy source for photosynthesis. As light intensity increases, the rate of photosynthesis generally increases as well, following a characteristic light response curve.
However, this relationship is not linear. At a certain point, the photosynthetic rate reaches a plateau, known as light saturation. Beyond this point, increasing light intensity no longer increases photosynthesis, and in some cases, can even lead to photoinhibition and damage to the photosynthetic apparatus.
Carbon Dioxide: Fueling the Calvin Cycle
Carbon dioxide (CO2) is the carbon source for glucose production in photosynthesis. Similar to light intensity, increasing CO2 concentration generally increases the rate of photosynthesis, up to a certain point.
The effect of CO2 concentration is particularly important in the context of rising atmospheric CO2 levels. While increased CO2 can initially boost photosynthesis, other factors, such as nutrient availability and water stress, can limit the extent of this stimulation.
Temperature: Finding the Optimal Range
Temperature affects the enzymatic reactions involved in photosynthesis. Every plant species has an optimal temperature range for photosynthesis.
Within this range, the rate of photosynthesis is maximized. Below this range, enzymatic activity slows down, and above it, enzymes can become denatured, leading to a decline in photosynthetic rate. Extreme temperatures can cause significant damage to plant tissues.
Water Availability: The Key to Stomatal Conductance
Water is essential for photosynthesis, not only as a reactant but also for maintaining turgor pressure in cells and facilitating gas exchange. Water stress can lead to stomatal closure.
Stomata are the pores on the leaf surface that regulate CO2 uptake and water loss. When water is scarce, plants close their stomata to conserve water, which also restricts CO2 entry and reduces photosynthetic rates.
Plant-Specific Factors: Internal Controls of Photosynthesis
Beyond the external environment, a plant's own characteristics can also influence its photosynthetic capacity. Leaf age, health, and nutrient availability each play a significant role.
Leaf Age and Health: A Tale of Development and Senescence
Leaf age significantly impacts photosynthetic efficiency. Young, developing leaves have lower photosynthetic rates compared to mature leaves.
As leaves age and enter senescence, their photosynthetic capacity declines due to chlorophyll degradation and decreased enzyme activity. Healthy leaves, free from disease and stress, generally exhibit higher photosynthetic rates.
Nutrient Availability: The Building Blocks of Photosynthesis
Nutrients, particularly nitrogen (N) and phosphorus (P), are essential for photosynthesis. Nitrogen is a key component of chlorophyll and photosynthetic enzymes.
Phosphorus is involved in energy transfer and the synthesis of essential molecules. Nutrient deficiencies can limit photosynthetic capacity and overall plant growth.
Stomatal Conductance: Regulating Gas Exchange
Stomata play a crucial role in regulating the exchange of gases between the plant and the atmosphere. Stomatal conductance, a measure of the rate at which gases can pass through the stomata, directly affects photosynthetic rates.
Stomata open and close in response to various environmental and internal signals, such as light intensity, CO2 concentration, water availability, and hormonal signals. By controlling CO2 entry and water loss, stomata act as gatekeepers of photosynthesis.
Having explored the factors that influence photosynthetic measurements, it's time to turn our attention to how this knowledge is applied in the real world. Measuring photosynthesis isn't just an academic exercise; it's a powerful tool with far-reaching implications for agriculture, forestry, environmental science, global change research, and plant physiology. Let's delve into the diverse applications of these measurements, demonstrating their practical importance in addressing some of the world's most pressing challenges.
Photosynthesis in Action: Real-World Applications of Measurement
The ability to accurately measure photosynthesis unlocks a wealth of insights across various disciplines. From optimizing crop yields to understanding ecosystem responses to climate change, photosynthetic measurements provide critical data for informed decision-making.
Agriculture: Enhancing Crop Productivity
Photosynthesis is the foundation of crop productivity. By measuring photosynthetic rates, farmers and researchers can gain valuable information for optimizing agricultural practices.
Irrigation and Fertilization Strategies
Photosynthetic measurements can inform irrigation strategies by indicating when plants are experiencing water stress, even before visible symptoms appear. This allows for timely irrigation, preventing yield losses.
Similarly, monitoring photosynthetic rates can help determine the optimal levels of fertilization. Over-fertilization can be costly and environmentally damaging, while under-fertilization can limit growth. By measuring photosynthesis, farmers can fine-tune nutrient application to maximize crop yields while minimizing environmental impact.
For example, an IRGA can be used to measure the CO2 uptake of a crop under different irrigation regimes. The data can then be used to determine the optimal watering schedule for maximizing photosynthetic efficiency and yield.
Forestry: Assessing Forest Health and Carbon Sequestration
Forests play a crucial role in the global carbon cycle. Measuring photosynthesis in forests is essential for assessing their health and their ability to sequester carbon dioxide from the atmosphere.
Monitoring Forest Responses to Environmental Changes
Photosynthetic measurements can be used to monitor the impacts of environmental stressors such as air pollution, drought, and insect infestations on forest health. A decline in photosynthetic rates can indicate that a forest is under stress and may be more vulnerable to further damage.
Chlorophyll fluorescence, for example, can be used to detect early signs of stress in trees, allowing for timely intervention to prevent widespread damage. Moreover, long-term monitoring of photosynthetic rates can provide valuable data on the effects of climate change on forest ecosystems.
Environmental Science: Unraveling Ecosystem Dynamics
Photosynthesis is a key process in almost all ecosystems, and understanding its dynamics is essential for comprehending how ecosystems function.
Understanding the Impact of Pollution on Plant Health
Pollutants such as ozone and sulfur dioxide can directly inhibit photosynthesis. By measuring photosynthetic rates in plants exposed to these pollutants, scientists can assess the extent of the damage and develop strategies for mitigating the impacts of pollution on plant health and ecosystem function.
Photosynthetic measurements can also be used to monitor the effectiveness of pollution control measures.
Global Change Research: Predicting Future Climate Scenarios
Accurate models are essential for predicting the impacts of climate change. Photosynthetic data is crucial for refining these models.
Improving Climate Models
Photosynthesis is a major driver of the global carbon cycle, and accurate photosynthetic data is essential for improving the accuracy of climate models. These models are used to predict future climate scenarios and to assess the potential impacts of climate change on ecosystems and human societies.
By incorporating accurate photosynthetic data into climate models, scientists can improve the reliability of their predictions and develop more effective strategies for mitigating the impacts of climate change.
Plant Physiology: Decoding the Mechanisms of Photosynthesis
Beyond its applied applications, photosynthesis research is fundamental to understanding the inner workings of plants.
Studying the Effects of Genetic Modifications
Photosynthetic measurements are invaluable tools for studying the effects of genetic modifications on photosynthetic pathways. By measuring photosynthetic rates in genetically modified plants, researchers can assess whether the modifications have improved or impaired photosynthetic efficiency.
This information can be used to develop new crop varieties with enhanced photosynthetic capacity, leading to increased yields and improved food security. In addition, these studies can help to elucidate the complex mechanisms that regulate photosynthesis, providing insights that can be used to improve crop production and ecosystem management.
Having explored the factors that influence photosynthetic measurements, it's time to turn our attention to how this knowledge is applied in the real world. Measuring photosynthesis isn't just an academic exercise; it's a powerful tool with far-reaching implications for agriculture, forestry, environmental science, global change research, and plant physiology. Let's delve into the diverse applications of these measurements, demonstrating their practical importance in addressing some of the world's most pressing challenges.
Pioneers of Photosynthesis: Honoring Key Scientists
The intricate understanding of photosynthesis we possess today is built upon the tireless work and groundbreaking discoveries of numerous scientists. Recognizing the contributions of these pioneers is essential to appreciating the scientific journey that has unlocked the secrets of how plants power life on Earth. Let's delve into the pivotal roles of a few key figures.
Hans Gaffron: Unveiling Adaptations in Photosynthesis
Hans Gaffron (1902-1979) was a German-American biochemist whose research significantly advanced our understanding of photosynthesis, particularly in algae. Gaffron's most notable contribution was the discovery of adaptations in photosynthetic organisms to varying light intensities.
He found that algae, when shifted from low to high light intensity, did not immediately reach their maximum photosynthetic rate. Instead, they underwent a period of adaptation, suggesting the existence of complex regulatory mechanisms within the photosynthetic machinery. This work highlighted the dynamic nature of photosynthesis. It demonstrated that the process isn't static but responds and adjusts to environmental cues.
Gaffron also explored the effects of hydrogen gas on algal metabolism. His work paved the way for future research into alternative photosynthetic pathways and the potential for biohydrogen production. His meticulous experimentation and insightful interpretations shaped the trajectory of photosynthesis research.
Melvin Calvin: Deciphering the Calvin Cycle
Melvin Calvin (1911-1997) was an American biochemist best known for elucidating the Calvin Cycle, also known as the light-independent reactions of photosynthesis. This cycle describes the process by which carbon dioxide is fixed and converted into glucose in plants.
Using radioactive carbon-14 as a tracer, Calvin and his team mapped the complex series of chemical reactions involved in carbon fixation. This groundbreaking work earned him the Nobel Prize in Chemistry in 1961.
The Calvin Cycle is a cornerstone of modern biology, providing a detailed understanding of how plants convert atmospheric carbon dioxide into the building blocks of life. Calvin's research revolutionized our understanding of photosynthesis and laid the foundation for advancements in agriculture and biotechnology. His legacy continues to inspire scientists to unravel the complexities of biochemical pathways.
Cornelius Van Niel: Unifying Photosynthetic Principles
Cornelius Van Niel (1897-1985) was a Dutch-American microbiologist who made fundamental contributions to our understanding of the chemistry of photosynthesis. He is best known for his work on photosynthetic bacteria, which utilize different electron donors than water.
Van Niel proposed a unifying equation for photosynthesis, stating that in green plants, algae, and cyanobacteria, water is the electron donor, while in photosynthetic bacteria, other compounds, such as hydrogen sulfide, can serve as electron donors. This insight led to a profound understanding of the general principles underlying photosynthesis.
His work demonstrated that oxygen produced during photosynthesis originates from water, not carbon dioxide, as previously believed. This discovery revolutionized our understanding of the process. Van Niel's emphasis on comparative biochemistry and his ability to synthesize disparate observations into a coherent framework had a lasting impact on the field of photosynthesis research. He was a visionary scientist whose work continues to shape our understanding of the fundamental processes of life.
Video: Measuring Photosynthesis: Ultimate Guide!
FAQs: Measuring Photosynthesis
Here are some frequently asked questions about measuring photosynthesis, designed to help you better understand the processes and techniques involved.
Why is measuring photosynthesis important?
Measuring photosynthesis helps us understand plant health, productivity, and how plants respond to environmental changes. This is crucial for agriculture, climate change research, and ecosystem management. Understanding the rate of measuring photosynthesis can help us optimize growing conditions.
What are the primary methods for measuring photosynthesis?
The main methods include measuring oxygen evolution, carbon dioxide uptake, and chlorophyll fluorescence. Each technique offers different insights into the photosynthetic process and can be used depending on the specific research goals. Measuring photosynthesis is central to understanding these processes.
What factors can affect measurements of photosynthesis?
Several factors can influence photosynthesis measurements, including light intensity, temperature, carbon dioxide concentration, water availability, and the plant's health. It's essential to control or account for these factors to obtain accurate and reliable results when measuring photosynthesis.
What is chlorophyll fluorescence and how is it used to measure photosynthesis?
Chlorophyll fluorescence measures the light energy re-emitted by chlorophyll molecules after light absorption. Changes in fluorescence indicate the efficiency of photosystem II, a key component of photosynthesis. Analyzing fluorescence helps scientists assess plant stress and the overall rate of measuring photosynthesis.