Mass Change Explained: Shocking Transformations!
The principles governing Physics significantly influence our understanding of Nuclear Reactions, both of which are foundational to comprehending change in mass. Observing phenomena at sites like CERN allows scientists to rigorously test theories regarding mass conservation and transformation. Such experiments are often influenced and interpreted through the contributions of prominent figures, for instance, the theoretical work of Albert Einstein, whose equations fundamentally link energy and mass. Therefore, understanding change in mass necessitates examining these interconnected entities and their respective attributes, offering a comprehensive perspective on the subject.
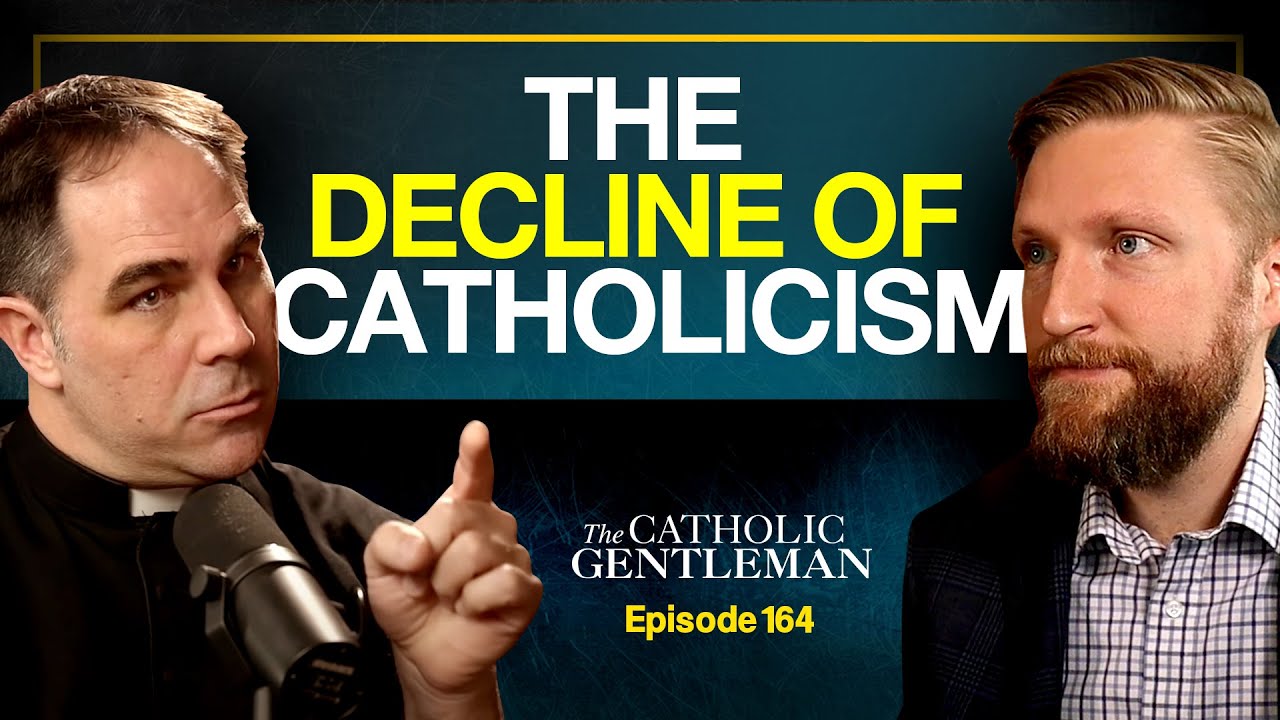
Image taken from the YouTube channel The Catholic Gentleman , from the video titled What Has to Change at Mass NOW w/ Fr. Donald Calloway .
Imagine the raw, untamed power of a nuclear explosion, a cataclysmic event that reshapes matter and releases unimaginable energy. Or consider the opposite: the elegant dance of particle creation within a supercollider, where energy coalesces into brand new particles with measurable mass. These seemingly disparate phenomena share a common thread: they are both dramatic examples of mass change, the central concept we'll be exploring.
But what exactly is mass, and why should we care about how it changes?
Defining Mass: The Foundation of Reality
At its most fundamental, mass is a measure of an object's resistance to acceleration. It is an intrinsic property, a fundamental quantity that dictates how much force is required to change an object's motion. A more massive object requires a greater force to accelerate at the same rate as a less massive one. This is why it's harder to push a car than a bicycle.
Mass is also the source of gravitational force, the force that binds planets to stars and keeps our feet firmly planted on the ground. Without mass, the universe as we know it simply wouldn't exist.
The Significance of Mass Change
The idea that mass can change may seem counterintuitive. After all, we tend to think of mass as a fixed, immutable characteristic of an object. However, modern science has revealed that mass is not always conserved. It can be altered through a variety of processes, ranging from nuclear reactions to the subtle shifts that occur during chemical reactions.
Understanding these changes is crucial for a number of reasons. It allows us to:
- Harness the power of nuclear energy.
- Develop new technologies for medicine and materials science.
- Probe the fundamental laws of physics at the subatomic level.
- Comprehend the evolution of the universe from its earliest moments.
Exploring the Landscape of Mass Change
This exploration will journey through the fascinating world of mass change, examining its various facets and unveiling the underlying principles that govern it. We will begin with the classical view, rooted in the Law of Conservation of Mass, pioneered by scientists like Antoine Lavoisier.
We will then delve into Einstein's revolutionary theory of mass-energy equivalence (E=mc²), which forever changed our understanding of the relationship between mass and energy. We'll explore how these principles manifest in nuclear reactions, where mass changes are most dramatic, and in the subtle shifts that occur even in ordinary chemical reactions.
Our journey will touch upon the insights gained from particle physics experiments, conducted at facilities like the Large Hadron Collider (LHC), which probe the very nature of mass at the subatomic level. By exploring these diverse areas, we aim to provide a comprehensive overview of mass change and its profound implications for our understanding of the universe.
Imagine the raw, untamed power of a nuclear explosion, a cataclysmic event that reshapes matter and releases unimaginable energy. Or consider the opposite: the elegant dance of particle creation within a supercollider, where energy coalesces into brand new particles with measurable mass. These seemingly disparate phenomena share a common thread: they are both dramatic examples of mass change, the central concept we'll be exploring.
But what exactly is mass, and why should we care about how it changes?
At its most fundamental, mass is a measure of an object's resistance to acceleration. It is an intrinsic property, a fundamental quantity that dictates how much force is required to change an object's motion.
A more massive object requires a greater force to accelerate at the same rate as a less massive one. This is why it's harder to push a car than a bicycle.
Mass is also the source of gravitational force, the force that binds planets to stars and keeps our feet firmly planted on the ground. Without mass, the universe as we know it simply wouldn't exist.
The idea that mass can change may seem counterintuitive. After all, we tend to think of mass as a fixed, immutable characteristic of an object.
However, modern science has revealed that mass is not always conserved. It can be altered through a variety of processes, ranging from nuclear reactions to the subtle shifts that occur during chemical reactions.
Understanding these changes is critical to unlocking a deeper understanding of the universe. But before we dive into the complexities of modern physics, it’s essential to appreciate the foundation upon which our understanding of mass was built: the Law of Conservation of Mass.
The Classical View: Law of Conservation of Mass
For centuries, the bedrock of chemical understanding rested firmly on the principle of mass conservation. This law, seemingly self-evident in everyday experience, states that mass is neither created nor destroyed in ordinary chemical reactions.
In simpler terms, if you start with 10 grams of reactants, you should end up with 10 grams of products, no matter how dramatic the transformation.
Defining the Law: Mass Invariance in Chemical Reactions
The Law of Conservation of Mass dictates that in a closed system, the total mass of the reactants before a chemical reaction is equal to the total mass of the products after the reaction.
This principle applies to traditional chemical reactions where atoms are rearranged through the breaking and forming of chemical bonds.
For example, if you burn wood, the mass of the wood and the oxygen consumed in the burning process will equal the mass of the ash, carbon dioxide, water vapor, and other byproducts released.
This understanding forms the basis for stoichiometry, the branch of chemistry that deals with the quantitative relationships between reactants and products in chemical reactions.
Antoine Lavoisier: The Father of Modern Chemistry
While the idea of conservation had been floated before, Antoine Lavoisier is widely credited with establishing the Law of Conservation of Mass as a fundamental principle of chemistry through rigorous experimentation.
Lavoisier, a French nobleman and chemist, meticulously measured the mass of reactants and products in various chemical reactions, particularly combustion.
His experiments demonstrated that mass was indeed conserved, even when substances underwent dramatic transformations.
Lavoisier's work revolutionized chemistry by shifting it from a qualitative to a quantitative science.
By emphasizing precise measurement and careful observation, he laid the groundwork for modern chemical analysis and the development of the periodic table.
Lavoisier's meticulous approach challenged existing theories and established a new standard for scientific inquiry.
The Law's Limitations: When Conservation Breaks Down
Despite its widespread applicability and historical significance, the Law of Conservation of Mass is not universally true.
It holds true for ordinary chemical reactions at everyday energy levels.
However, when we venture into the realm of nuclear reactions or relativistic physics, the law begins to show its limitations.
At extremely high energies, such as those found in nuclear reactions or particle accelerators, mass can be converted into energy and vice versa, as described by Einstein's famous equation, E=mc².
In these scenarios, the combined mass and energy of a system are conserved, but mass itself is not.
This doesn't negate the importance of Lavoisier's work; rather, it highlights the fact that scientific laws are often approximations that are valid within certain limits.
The discovery of the limitations of the Law of Conservation of Mass paved the way for a deeper understanding of the relationship between mass and energy.
It forced scientists to rethink their assumptions about the nature of matter and the universe itself, opening up new avenues of scientific exploration.
But the story doesn’t end with Lavoisier. The classical view, while powerful in its domain, proved to be an approximation. A deeper understanding of mass awaited a revolution – a revolution sparked by a young patent clerk named Albert Einstein.
Einstein's Revolution: Mass-Energy Equivalence
Albert Einstein irrevocably altered our understanding of the universe, and with it, the very definition of mass. His theories, particularly special relativity, unveiled a profound connection between mass and energy, forever changing how we perceive these fundamental quantities.
Unveiling Relativity: A Prelude to E=mc²
To fully grasp the significance of mass-energy equivalence, it's essential to touch upon Einstein's theory of special relativity. This theory, published in 1905, introduced revolutionary concepts about space, time, and the speed of light.
One of the core tenets of special relativity is that the laws of physics are the same for all observers in uniform motion. This seemingly simple statement had far-reaching consequences, ultimately leading to the realization that mass and energy are not independent but rather two sides of the same coin.
Decoding E=mc²: The Universe's Most Famous Equation
At the heart of Einstein's revolution lies his most famous equation: E=mc². This deceptively simple formula encapsulates a profound truth about the nature of reality.
E represents energy, m represents mass, and c represents the speed of light in a vacuum – an astonishing 299,792,458 meters per second. The equation states that energy and mass are interchangeable, and the conversion factor is the square of the speed of light.
This means that a small amount of mass can be converted into an enormous amount of energy, and vice versa. The sheer magnitude of c² explains why even tiny changes in mass can result in substantial energy releases.
Mass and Energy: Two Sides of the Same Coin
Einstein's equation reveals that mass is not simply a measure of an object's resistance to acceleration; it is also a form of stored energy. When an object gains energy, it also gains mass, albeit often by an imperceptibly small amount in everyday scenarios.
Conversely, when an object loses energy, it loses mass. This interchangeability is the essence of mass-energy equivalence.
It’s no longer appropriate to consider mass as an immutable property. Energy and mass are intertwined, dynamic quantities.
Real-World Manifestations: Examples of Mass-Energy Conversion
The implications of E=mc² are not merely theoretical; they are evident in a wide range of phenomena, from the devastating power of nuclear weapons to the life-giving energy of the sun.
Nuclear Weapons
Perhaps the most dramatic demonstration of mass-energy conversion is the nuclear weapon. In a nuclear explosion, a small amount of nuclear material undergoes fission, splitting into smaller atoms.
The total mass of the resulting fragments is slightly less than the mass of the original atom. This "missing" mass is converted into an enormous amount of energy, released as heat, light, and radiation.
The Sun's Energy Production
The sun, the source of nearly all energy on Earth, operates on the principle of nuclear fusion. Deep within the sun's core, hydrogen atoms fuse together to form helium atoms.
Again, the mass of the resulting helium atom is slightly less than the combined mass of the original hydrogen atoms. This mass difference is converted into energy, which radiates outward from the sun, providing light and warmth to our planet. The sun is continuously losing mass as it shines.
Particle Physics
Another compelling example is found within particle accelerators like the Large Hadron Collider (LHC). Scientists accelerate particles to near-light speed and collide them.
These collisions can create new, heavier particles. The extra mass of these new particles comes directly from the kinetic energy of the colliding particles, once again demonstrating the principle of mass-energy equivalence.
But the true magnitude of mass change becomes strikingly apparent when we delve into the realm of nuclear reactions. Here, within the heart of atoms, forces far greater than those governing chemical bonds come into play. It is in these transformations, where the very identity of elements can be altered, that mass undergoes dramatic shifts, offering compelling validation of Einstein’s revolutionary insights.
Nuclear Transformations: Where Mass Changes Dramatically
Nuclear reactions represent a departure from the relatively stable world of chemical reactions. Instead of simply rearranging atoms, nuclear reactions alter the composition of the nucleus itself, the atom's core. These alterations are accompanied by significant energy releases and, crucially, measurable changes in mass.
The Nucleus: A Realm of Immense Energy
The nucleus houses protons and neutrons, collectively known as nucleons, bound together by the strong nuclear force. This force is immensely powerful, far exceeding the electromagnetic forces that govern chemical bonding. When the configuration of nucleons is altered, such as in fission or fusion, tremendous amounts of energy are released, accompanied by a corresponding change in mass, as predicted by E=mc².
Nuclear Reactions as Mass Change Source
Unlike chemical reactions, where mass is effectively conserved, nuclear reactions demonstrate a clear and measurable difference between the mass of the reactants and the mass of the products. This difference, albeit small in absolute terms, is significant because it's directly converted into energy.
It’s a direct, practical application of E=mc².
Mass Defect
The mass change in nuclear reactions is often described as the "mass defect." This defect represents the portion of the original mass converted into energy during the reaction. The magnitude of the mass defect, and thus the energy released, depends on the specific nuclear reaction and the isotopes involved.
Nuclear Fission
Nuclear fission is the process where a heavy nucleus, like uranium-235, splits into two or more smaller nuclei. This splitting is typically induced by the absorption of a neutron.
The resulting fragments have a combined mass slightly less than the original nucleus and neutron, with the "missing" mass converted into kinetic energy of the fragments and released as heat and radiation.
Fission and Nuclear Power
Nuclear power plants harness the energy released during controlled nuclear fission. In these reactors, the fission of uranium-235 or plutonium-239 generates heat, which is used to produce steam, drive turbines, and generate electricity.
Nuclear Fusion
In contrast to fission, nuclear fusion involves the combining of two light nuclei to form a heavier nucleus. This process releases even greater amounts of energy per unit mass than fission.
The most well-known example is the fusion of hydrogen isotopes in the sun.
Fusion in Stars
The sun's energy originates from the nuclear fusion of hydrogen into helium. This process, occurring at extremely high temperatures and pressures in the sun's core, releases vast quantities of energy that sustain life on Earth.
Fusion Reactors: The Future of Energy?
Scientists are actively pursuing the development of fusion reactors on Earth. Achieving sustained and controlled fusion is a formidable challenge, but the potential rewards – a clean, abundant, and virtually inexhaustible energy source – are immense.
Radioactive Decay
Radioactive decay is a spontaneous process in which an unstable nucleus transforms into a more stable configuration by emitting particles or energy. This transformation always results in a change of mass. There are several types of radioactive decay:
- Alpha Decay: Emission of an alpha particle (helium nucleus). This decreases the mass number by 4 and the atomic number by 2.
- Beta Decay: Emission of a beta particle (electron or positron). Beta decay doesn't significantly alter the mass number, but it changes the atomic number by 1.
- Gamma Decay: Emission of a gamma ray (high-energy photon). Gamma decay doesn't change the mass number or atomic number; it simply lowers the energy of the nucleus.
Mass Changes in Radioactive Decay
In each type of radioactive decay, the total mass of the daughter nucleus and emitted particles is less than the mass of the original nucleus. This mass difference is converted into the kinetic energy of the emitted particles and gamma rays.
Isotopes
Isotopes are variants of a chemical element which differ in neutron number, and consequently in nucleon number. All isotopes of a given element have the same number of protons but different numbers of neutrons in each atom. While the chemical properties of different isotopes of an element are almost identical, their nuclear properties can vary significantly.
Isotopes and Radioactive Decay
Some isotopes are stable, while others are radioactive. Radioactive isotopes undergo radioactive decay, transforming into different isotopes or elements. The type of decay and the rate of decay (half-life) are specific to each radioactive isotope.
The decay of a radioactive isotope will therefore change its mass and potentially change the element it is.
Subatomic World: Exploring Mass Change with Particle Physics
The world of particle physics takes us to the smallest scales of existence, where the rules governing mass and energy become even more profound. Here, we don't just observe the transformation of existing particles; we witness the creation of new ones from pure energy, and the reverse process, annihilation, where particles disappear, converting back into energy. This realm offers the most direct and compelling validation of Einstein's E=mc², revealing the intimate relationship between mass and energy.
Particle Physics Insights: Unveiling the Infinitesimal
Particle physics experiments are designed to probe the fundamental building blocks of matter and their interactions. By colliding particles at extremely high energies, physicists can create conditions similar to those that existed in the early universe, moments after the Big Bang.
These high-energy collisions can produce a shower of new particles, some of which are fleeting and exotic. Observing these events provides invaluable insights into the nature of mass, the forces that govern the subatomic world, and the fundamental laws of physics.
The Large Hadron Collider (LHC): A Window into Mass Transformation
The Large Hadron Collider (LHC) at CERN is a monumental instrument built to explore the frontiers of particle physics. This massive machine accelerates particles to near the speed of light and smashes them together, recreating conditions not seen since the universe's earliest moments.
The LHC allows scientists to study the products of these collisions with unprecedented precision, enabling them to observe the creation of new particles, measure their properties, and test the predictions of the Standard Model of particle physics.
Creating Mass from Energy
One of the most significant achievements of the LHC has been the discovery of the Higgs boson. This particle, predicted decades ago, is responsible for giving mass to other fundamental particles through the Higgs field.
The LHC provides direct evidence of mass creation by converting kinetic energy into mass, according to E=mc². When two protons collide at high energy, their kinetic energy can be converted into the mass of newly formed particles.
Studying Particle Decay and Mass
The LHC also enables physicists to study the decay of unstable particles. By precisely measuring the mass and decay products of these particles, they can gain further insights into the fundamental forces and interactions that govern the subatomic world.
The LHC's experiments offer a unique opportunity to test our understanding of mass and energy, explore new physics beyond the Standard Model, and potentially uncover new dimensions and particles that could revolutionize our understanding of the universe.
Subtle Shifts: Mass Changes in Chemical Reactions
Having explored the dramatic mass transformations within nuclear reactions and the subatomic world, it's easy to assume that mass change is exclusive to these high-energy realms. However, mass also undergoes subtle, yet measurable, shifts during ordinary chemical reactions. Though not as pronounced as nuclear events, these changes offer a deeper understanding of the intricate relationship between mass, energy, and chemical bonding.
The Atomic Dance: Mass Fluctuations in Chemical Bonds
At the atomic level, chemical reactions involve the breaking and formation of chemical bonds. These bonds are not simply static connections, but rather represent potential energy stored within the electron configurations of atoms.
When a chemical reaction releases energy (an exothermic reaction), some of that energy originates from a slight decrease in the mass of the reacting system. Conversely, when a reaction requires energy input to proceed (an endothermic reaction), there is a minute increase in the mass of the system as energy is absorbed.
This is a direct consequence of Einstein's famous equation, E=mc².
Quantifying the Immeasurable: Detecting Subtle Mass Changes
While the mass changes in chemical reactions are incredibly small, they are theoretically measurable with sufficiently precise instruments. The energy released or absorbed during a reaction (enthalpy change, ΔH) is directly related to the mass change (Δm) via E=mc², where ΔE = Δmc².
In practice, detecting these minute mass differences is extremely challenging due to their infinitesimal scale. For example, even in a highly exothermic reaction, the mass change might be on the order of picograms (10⁻¹² grams) or less, making direct measurement exceedingly difficult.
The Heat-Mass Connection: Energy Transfer and Mass Equivalence
The relationship between mass changes, heat, and energy in chemical reactions is fundamental. Exothermic reactions, which release heat, undergo a minuscule decrease in mass.
This decrease arises because some of the mass is converted into the kinetic energy of the molecules, which we perceive as heat.
Endothermic reactions, conversely, absorb heat from their surroundings, and this absorbed energy manifests as a tiny increase in the mass of the system.
Think of it as energy being "borrowed" from the environment, which results in a corresponding increase in the system's mass, albeit an extremely subtle one.
Implications and Practical Considerations
While these mass changes in chemical reactions are generally negligible for most practical purposes, their existence underscores a crucial principle: mass and energy are fundamentally intertwined.
Even seemingly "ordinary" chemical processes are governed by the same laws that dictate the behavior of nuclear reactions and the subatomic world. Understanding this connection reinforces the power and universality of Einstein's mass-energy equivalence, highlighting its relevance across all scales of scientific inquiry.
Video: Mass Change Explained: Shocking Transformations!
Mass Change Explained: Frequently Asked Questions
What exactly does "mass change" mean in this context?
"Mass change" refers to any alteration in the amount of matter that makes up an object or system. This change in mass can occur due to various processes, such as the addition or removal of material, or even through energy conversion.
What are some common examples of mass change transformations?
Examples include burning wood (mass decreases as it turns into ash and gases), a plant growing (mass increases as it absorbs water and nutrients), or a nuclear reaction in a reactor (a tiny bit of mass is converted into enormous energy). These all demonstrate how change in mass can be observed.
Is change in mass always a visible or dramatic event?
No, change in mass can be very subtle. For example, a sealed container gaining a microscopic layer of dust over many years experiences a change in mass, even though it might not be immediately noticeable.
Does the type of matter affect the possibility of a mass change?
All matter is subject to potential mass changes. The ease and method of achieving a change in mass depends greatly on the type of matter. For example, liquids might experience loss or gain easier compared to solids, but change in mass will be there.