Isopentyl Acetate IR: Spectroscopy Guide
Infrared (IR) spectroscopy serves as a pivotal analytical technique, which leverages the vibrational modes of molecules to elucidate their structure and composition. Characterization of isopentyl acetate ir spectra provides essential data, where isopentyl acetate, commonly synthesized in organic chemistry labs, exhibits characteristic absorption bands corresponding to its ester functional group. Interpretation of these spectra often involves comparison with spectral databases maintained by organizations like the National Institute of Standards and Technology (NIST). Moreover, computational chemistry tools, such as those employing Density Functional Theory (DFT), can predict IR spectra, aiding in the accurate assignment of experimental isopentyl acetate ir peaks.
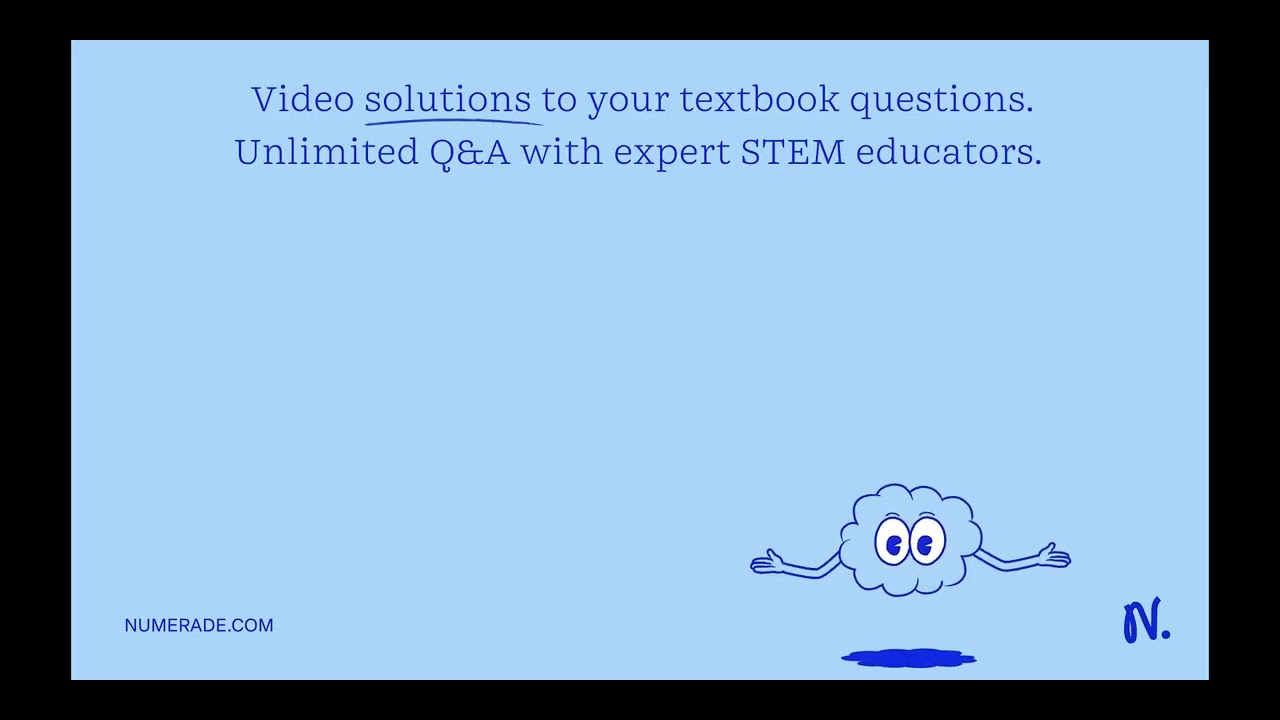
Image taken from the YouTube channel Study STEM With Numerade , from the video titled List three major peaks found in the Infrared spectrum of Isopentyl Acetate (Banana Oil) and the bon… .
Unveiling Isopentyl Acetate Through Infrared Light
Isopentyl acetate, also known as isoamyl acetate, is a volatile ester renowned for its distinctive banana-like aroma. This compound finds extensive applications across diverse industries, from food and beverage to cosmetics and pharmaceuticals.
Its primary role lies in artificial flavoring, imparting the characteristic scent to candies, baked goods, and various fruit-flavored products. Beyond flavor enhancement, isopentyl acetate serves as a solvent for lacquers and varnishes, as well as a component in certain cleaning agents.
The Analytical Power of Infrared Spectroscopy
Infrared (IR) spectroscopy stands as a potent analytical technique, enabling the identification and characterization of molecules based on their vibrational properties. This method leverages the interaction between infrared radiation and molecular bonds to generate a unique spectral fingerprint.
This fingerprint provides invaluable insights into a molecule's structure and composition. By analyzing the absorption patterns within an IR spectrum, researchers and analysts can determine the presence of specific functional groups, assess purity, and even quantify the concentration of a target compound.
Why IR Spectroscopy for Isopentyl Acetate?
For those working with isopentyl acetate, a robust understanding of IR spectroscopy is not merely beneficial, but essential. This analytical tool provides a rapid and non-destructive means of verifying the identity and quality of this important ester.
Whether you're involved in flavor formulation, solvent production, or chemical research, IR spectroscopy offers a precise and reliable method for characterizing isopentyl acetate. By mastering the principles and applications of this technique, you can gain a deeper understanding of this compound's behavior and ensure the integrity of your products and processes.
Scope of this Guide
This guide provides a comprehensive exploration of isopentyl acetate analysis using IR spectroscopy. From fundamental principles to practical applications, we aim to equip you with the knowledge and skills necessary to confidently interpret IR spectra and leverage this technique for your specific needs.
We will delve into the molecular structure of isopentyl acetate, the theoretical underpinnings of IR spectroscopy, and the practical aspects of sample preparation and spectral interpretation. By the end of this guide, you will be well-versed in the art and science of unveiling isopentyl acetate through infrared light.
Isopentyl Acetate: A Molecular Overview
To fully leverage IR spectroscopy in the analysis of isopentyl acetate, it's crucial to first establish a firm understanding of its molecular structure. This foundational knowledge will serve as the bedrock for interpreting the spectral features observed later.
Decoding the Chemical Structure
Isopentyl acetate, chemically represented as CH3COOCH2CH2CH(CH3)2, is an ester derived from acetic acid and isoamyl alcohol (isopentanol). At its core lies an acetyl group (CH3CO-) bonded to an isopentyl group (CH3)2CHCH2CH2-. This arrangement gives rise to its characteristic chemical and physical properties.
The molecule is composed of carbon, hydrogen, and oxygen atoms strategically arranged to form distinct functional groups. Recognizing these functional groups is paramount for IR spectral analysis.
Key Functional Groups and Their Significance
The isopentyl acetate molecule hosts several key functional groups that exhibit distinct IR absorption characteristics. These include:
-
Carbonyl Group (C=O): This is arguably the most prominent functional group in isopentyl acetate. The carbonyl group is responsible for a strong absorption band in the region of 1720-1750 cm-1 in the IR spectrum. Its presence is a defining feature of esters.
-
C-H Bonds: Multiple C-H bonds are present throughout the molecule. These bonds, stemming from both the acetyl and isopentyl groups, give rise to absorption bands in the 2850-3000 cm-1 region, corresponding to C-H stretching vibrations.
-
Ester Linkage (C-O): The ester linkage, connecting the acetyl and isopentyl fragments, contains C-O bonds. These bonds contribute to absorption bands in the 1000-1300 cm-1 region, reflecting C-O stretching vibrations.
Functional Groups as Spectral Determinants
The significance of these functional groups stems from their ability to interact with infrared radiation in a predictable manner. When exposed to IR radiation, these bonds vibrate at specific frequencies.
These frequencies correspond to the energy of the IR radiation absorbed. Consequently, each functional group generates a unique absorption band in the IR spectrum.
By identifying the presence and position of these absorption bands, we can gain valuable insights into the composition and structure of isopentyl acetate. The distinct spectral signatures enable qualitative and quantitative analysis of the compound.
The Principles of Infrared Spectroscopy: A Foundation for Understanding
To fully appreciate the analytical power of IR spectroscopy, a grasp of its fundamental principles is essential. This section serves as a primer, demystifying the interaction between molecules and infrared radiation, and laying the groundwork for spectral interpretation.
Vibrational Spectroscopy: A Broader Perspective
Infrared (IR) spectroscopy is a subset of a larger field known as vibrational spectroscopy. This field encompasses techniques that probe the vibrational modes of molecules to glean information about their structure and dynamics. Other techniques in this area include Raman spectroscopy and inelastic neutron scattering.
IR spectroscopy specifically focuses on vibrational transitions that are accompanied by a change in the dipole moment of the molecule. This selection rule dictates which vibrations are "IR active" and will be observed in the spectrum.
Molecular Absorption of Infrared Radiation
At the heart of IR spectroscopy lies the phenomenon of molecules absorbing infrared radiation at specific frequencies. This absorption is quantized, meaning that a molecule will only absorb radiation that corresponds precisely to the energy required to transition between vibrational energy levels.
The specific frequencies at which a molecule absorbs are dictated by its molecular structure, the masses of the atoms, and the strength of the chemical bonds. When a molecule absorbs IR radiation of the correct frequency, it undergoes a vibrational excitation, resulting in a peak or band in the IR spectrum.
Normal Modes of Vibration: Stretching and Bending
Molecules do not simply vibrate in a random, chaotic manner. Instead, they vibrate in a set of specific patterns called normal modes of vibration. These modes can be broadly classified into two categories: stretching and bending.
Stretching Vibrations
Stretching vibrations involve changes in the bond length between two atoms. These are typically higher in frequency compared to bending vibrations.
For example, a carbonyl group (C=O) exhibits a characteristic stretching vibration that is highly sensitive to its chemical environment. Similarly, the C-H bonds in organic molecules exhibit stretching vibrations that are diagnostic of the type of carbon atom to which they are attached (e.g., sp3, sp2, or sp hybridized).
Bending Vibrations
Bending vibrations involve changes in the bond angle between three or more atoms. Bending vibrations generally require less energy than stretching vibrations, so they appear at lower wavenumbers in the IR spectrum.
Common examples of bending vibrations include scissoring, rocking, wagging, and twisting motions. These vibrations are particularly important in the fingerprint region of the IR spectrum, where they contribute to the unique spectral signature of a molecule.
Functional Groups and IR Absorption: Deciphering the Spectrum
[The Principles of Infrared Spectroscopy: A Foundation for Understanding To fully appreciate the analytical power of IR spectroscopy, a grasp of its fundamental principles is essential. This section serves as a primer, demystifying the interaction between molecules and infrared radiation, and laying the groundwork for spectral interpretation. Vibrat...]
The true power of infrared (IR) spectroscopy lies in its ability to identify the specific functional groups present within a molecule. This capability stems from the direct correlation between a functional group's structure and its characteristic absorption of infrared radiation. By carefully examining the absorption bands in an IR spectrum, we can deduce the presence or absence of key functional groups. This information provides valuable insights into the molecular composition of the sample.
The Direct Correlation Between Functional Groups and Absorption Frequencies
The absorption of IR radiation by a molecule is not a random event. Instead, it is a highly specific process dictated by the molecule's vibrational modes. Each functional group possesses a unique set of vibrational modes that are sensitive to the group's atomic masses, bond strengths, and overall geometry.
As a result, specific functional groups absorb IR radiation at predictable frequencies, leading to characteristic absorption bands in the IR spectrum. This one-to-one relationship is the foundation of spectral interpretation. For instance, carbonyl groups (C=O) consistently exhibit strong absorption bands in the range of 1650-1800 cm-1, making them readily identifiable.
Common Functional Groups and Their Typical IR Absorption Ranges
To effectively interpret IR spectra, it is essential to have a reference guide of common functional groups and their typical absorption ranges.
Functional Group | Typical IR Absorption Range (cm-1) | Intensity | Notes |
---|---|---|---|
O-H (alcohol) | 3200-3600 | Strong, Broad | Hydrogen bonding broadens the peak |
N-H (amine) | 3300-3500 | Medium, Broad | One or two peaks depending on the amine type |
C-H (alkane) | 2850-3000 | Medium | |
C=O (carbonyl) | 1650-1800 | Strong | Position varies with the specific carbonyl compound (e.g., ketone, ester, amide) |
C=C (alkene) | 1620-1680 | Medium | |
C≡C (alkyne) | 2100-2260 | Weak to Medium | |
C-O (ether, ester, alcohol) | 1000-1300 | Strong | Multiple bands are often present |
NO2 (nitro) | 1500-1600 & 1300-1380 | Strong | Two strong bands |
Note: The exact position of an absorption band can be influenced by factors such as the surrounding molecular environment and the physical state of the sample.
This table serves as a starting point for spectral interpretation, but careful consideration should be given to the intensity and shape of the bands. Additionally, the presence of multiple functional groups can lead to overlapping or shifted absorption bands, requiring careful analysis.
Case Studies: Examples of Functional Group Contributions
Consider the IR spectrum of ethanol (CH3CH2OH). We would expect to see prominent absorption bands corresponding to the O-H stretch (around 3200-3600 cm-1), C-H stretches (around 2850-3000 cm-1), and C-O stretch (around 1000-1300 cm-1). The presence and positions of these bands would provide strong evidence for the presence of an alcohol functional group in the molecule.
Similarly, in the IR spectrum of acetone (CH3COCH3), the most prominent feature is the strong absorption band due to the carbonyl group (C=O) at around 1715 cm-1. This band confirms the presence of a ketone functional group. The C-H stretches from the methyl groups would also be present, but the carbonyl peak is the most distinctive.
By carefully examining the IR spectrum and correlating the absorption bands with the known vibrational frequencies of functional groups, we can unlock a wealth of information about the molecular composition of a sample. This powerful analytical technique finds applications in diverse fields, including chemistry, materials science, and environmental monitoring.
Understanding Wavenumber and Transmittance/Absorbance: Key Spectral Parameters
Understanding the language of an IR spectrum requires familiarity with its fundamental parameters: wavenumber, transmittance, and absorbance. These concepts provide the framework for interpreting the data and extracting meaningful information about molecular composition and concentration.
Wavenumber: A Measure of Vibrational Energy
Wavenumber, expressed in reciprocal centimeters (cm-1), is the most common unit used to represent the position of absorption bands in an IR spectrum. It is directly proportional to the frequency of the infrared radiation absorbed by the molecule during vibrational transitions. Higher wavenumbers correspond to higher energy vibrations.
The relationship between wavenumber (ν̃), frequency (ν), and the speed of light (c) is given by:
ν̃ = ν / c
This means that each peak on the spectrum refers to the specific frequency of a molecular vibration. It is also used as a proxy for the vibrational energy. This is because energy is directly proportional to frequency (E = hν, where h is Planck's constant).
Transmittance and Absorbance: Gauging Light Interaction
Transmittance (T) and absorbance (A) quantify how infrared light interacts with the sample.
Transmittance is the fraction of incident light that passes through the sample. Expressed as a percentage, a high transmittance value indicates that most of the light at a given wavenumber passed through the sample unabsorbed.
Absorbance, on the other hand, is a measure of the light that is absorbed by the sample. It is logarithmically related to transmittance:
A = -log10(T)
High absorbance values signify strong absorption of light at that wavenumber. Absorbance is often preferred for quantitative analysis because it is directly proportional to the concentration of the absorbing species, per the Beer-Lambert Law.
The IR Spectrum: A Visual Representation
An IR spectrum is a graph that plots either transmittance or absorbance against wavenumber. Traditionally, IR spectra are displayed with wavenumber on the x-axis and transmittance on the y-axis.
In this format, peaks pointing downwards represent absorption bands, indicating that the molecule is absorbing infrared radiation at those specific wavenumbers.
Conversely, spectra can also be plotted with absorbance on the y-axis. In this case, peaks pointing upwards represent absorption bands, directly indicating the degree of light absorbed at each specific wavenumber.
Applying the Beer-Lambert Law for Quantitative Analysis
The Beer-Lambert Law is a cornerstone of quantitative spectroscopy. It establishes a direct relationship between absorbance (A), concentration (c), path length (l), and molar absorptivity (ε):
A = εcl
Where:
- A is the absorbance.
- ε is the molar absorptivity (a measure of how strongly a chemical species absorbs light at a given wavelength).
- c is the concentration of the analyte.
- l is the path length of the light beam through the sample.
In the context of isopentyl acetate analysis, the Beer-Lambert Law enables the determination of its concentration in a sample by measuring the absorbance at a characteristic wavenumber. Accurate quantitative analysis relies on careful calibration and adherence to the law's assumptions, such as a linear relationship between absorbance and concentration.
Deviations from linearity can occur at high concentrations or due to instrumental factors, necessitating careful method validation.
FT-IR Instrumentation: A Look Inside the Spectrometer
Understanding Wavenumber and Transmittance/Absorbance: Key Spectral Parameters transitions us to the instrumentation that measures these parameters.
An FT-IR spectrometer is not merely a "black box," but a sophisticated instrument built upon carefully selected components working in synergy. To effectively utilize and interpret FT-IR data, understanding these components is crucial. Let's explore the inner workings of the FT-IR spectrometer, shedding light on its core components and their functions.
The Basic Principles of FT-IR Spectrometry
Fourier-Transform Infrared (FT-IR) spectrometry, unlike its dispersive predecessor, acquires an entire spectrum simultaneously. This is achieved using an interferometer, most commonly a Michelson interferometer.
The core principle relies on splitting a beam of infrared light into two paths: one directed to a fixed mirror and the other to a moving mirror. The beams are then recombined, creating an interference pattern that varies depending on the position of the moving mirror.
This interference pattern, called an interferogram, contains information about all the frequencies of infrared light from the source. A mathematical transformation, the Fourier transform, is then applied to the interferogram to extract the spectrum: intensity as a function of wavenumber.
Infrared Sources: Illuminating the Molecular World
The infrared source is the heart of the FT-IR spectrometer, responsible for generating the infrared radiation used to probe the sample. The ideal source exhibits a broad, stable, and intense emission across the infrared region.
Several types of sources are employed, each with its own characteristics:
Globar Source
The globar is a commonly used source, consisting of a silicon carbide rod heated to a high temperature (typically 1000-1300 °C) by electrical resistance. It emits a continuous spectrum in the mid-infrared region, making it suitable for a wide range of applications.
Globar sources are known for their stability and long lifetime. However, they require significant power input and can be susceptible to thermal shock.
Mercury-Cadmium Telluride (MCT) Source
MCT sources use a semiconductor material to generate infrared radiation through photoluminescence. They typically offer higher intensity compared to globar sources, particularly at longer wavelengths.
MCT sources operate at lower temperatures, typically requiring liquid nitrogen cooling. They are often preferred for applications demanding high sensitivity or when analyzing weak absorbers.
Other Infrared Sources
Other sources, such as tungsten lamps or nichrome wires, may be used in specific applications, particularly in the near-infrared region. Their use is often dictated by specific wavelength requirements and cost considerations.
Infrared Detectors: Capturing the Molecular Response
The infrared detector is the component that senses the infrared radiation after it has interacted with the sample. The detector's role is to convert the infrared energy into an electrical signal that can be processed to create the IR spectrum.
The sensitivity and response characteristics of the detector are crucial for obtaining high-quality data. Several detector types are available:
Deuterated Triglycine Sulfate (DTGS) Detector
The DTGS detector is a pyroelectric detector that measures changes in temperature induced by the incident infrared radiation. It's a robust and versatile detector, commonly used in general-purpose FT-IR spectrometers.
DTGS detectors operate at room temperature and offer a relatively broad spectral response. However, they may exhibit lower sensitivity compared to other types of detectors.
Mercury-Cadmium Telluride (MCT) Detector
Similar to MCT sources, MCT detectors utilize semiconductor materials that are sensitive to infrared radiation. They operate by measuring changes in electrical conductivity upon exposure to infrared light.
MCT detectors offer significantly higher sensitivity compared to DTGS detectors, making them ideal for applications involving trace analysis or weakly absorbing samples. However, they require cryogenic cooling (typically liquid nitrogen) to operate effectively, adding to the instrument's complexity and cost.
Cooled vs. Uncooled Detectors
The choice between cooled and uncooled detectors often depends on the specific application. Cooled detectors, like MCT, offer superior sensitivity, whereas uncooled detectors, like DTGS, offer convenience and reduced operational costs.
Advantages of FT-IR over Dispersive IR Spectrometers
The advent of FT-IR spectrometry marked a significant advancement over traditional dispersive IR techniques. FT-IR offers several key advantages:
Fellgett's Advantage (Multiplex Advantage)
FT-IR spectrometers acquire data for all frequencies simultaneously, resulting in a significant increase in signal-to-noise ratio compared to dispersive instruments, which scan each frequency sequentially.
Jacquinot's Advantage (Throughput Advantage)
FT-IR spectrometers typically have larger apertures and lack the slits required in dispersive instruments. This results in higher energy throughput, leading to improved sensitivity and faster data acquisition.
Connes' Advantage (Wavenumber Accuracy)
FT-IR spectrometers use a laser as an internal standard, providing exceptional wavenumber accuracy and precision. This allows for more reliable spectral comparisons and library searching.
These advantages collectively make FT-IR the dominant technique in modern infrared spectroscopy, offering superior performance and versatility for a wide range of applications.
Sample Preparation Techniques for IR Spectroscopy: Optimizing Your Analysis
Understanding Wavenumber and Transmittance/Absorbance: Key Spectral Parameters transitions us to the instrumentation that measures these parameters.
However, even with the best instrumentation, poor sample preparation can undermine the accuracy and reliability of IR spectroscopic analysis.
Therefore, we now turn our attention to the critical role of proper sample preparation in obtaining high-quality IR spectra. The method selected depends heavily on the sample's physical state (solid, liquid, or gas) and its chemical properties.
The Importance of Proper Sample Preparation
The quality of an IR spectrum hinges significantly on how well the sample is prepared. Inadequate preparation can lead to several issues, including:
-
Scattering: Particles that are too large relative to the IR wavelength can scatter radiation, leading to a noisy baseline and reduced spectral resolution.
-
Distortion: Non-uniform sample thickness can cause distortions in peak intensities and band shapes.
-
Interference: Contaminants or impurities introduced during preparation can mask or alter the true spectral features of the analyte.
-
Inaccurate Results: The above factors can lead to quantitative or qualitative misinterpretations.
Ultimately, careful sample preparation is not merely a procedural step; it is fundamental to ensuring that the resulting spectrum accurately represents the sample's chemical composition and structure.
Sample Holders and Cells: An Overview
A range of sample holders and cells are available for IR spectroscopy, each designed to accommodate specific sample types and experimental requirements. The choice of holder impacts the path length of the IR beam through the sample, influencing the signal strength and spectral resolution.
Liquid Cells: Analyzing Liquid Samples
Liquid cells are designed to hold liquid samples in the path of the IR beam. These cells typically consist of two IR-transparent windows (e.g., NaCl, KBr, CaF2) separated by a thin spacer.
Considerations for Liquid Samples
When using liquid cells, several factors must be considered:
-
Path Length: The path length should be optimized to achieve adequate absorbance without saturation. Typical path lengths range from 0.01 mm to 1 mm.
-
Solvent Selection: The solvent should be IR-transparent in the region of interest and compatible with the cell window material.
- Common solvents include carbon tetrachloride (CCl4) and chloroform (CHCl3), although deuterated solvents are preferable.
- Deuterated solvents provide a less complex spectrum and don't obscure parts of the analyte spectrum.
- Common solvents include carbon tetrachloride (CCl4) and chloroform (CHCl3), although deuterated solvents are preferable.
-
Cell Filling: The cell should be filled carefully to avoid air bubbles, which can interfere with the IR beam.
Advantages and Disadvantages
Liquid cells offer the advantage of simplicity for analyzing liquid samples, however they present challenges in terms of solvent interference, potential for leaks, and limited applicability to strongly absorbing liquids.
KBr Pellets: Preparing Solid Samples
The KBr pellet technique is a common method for analyzing solid samples. It involves grinding the sample with potassium bromide (KBr), an IR-transparent salt, and compressing the mixture into a transparent pellet.
Procedure
The procedure involves:
- Finely grinding the solid sample to reduce particle size.
- Mixing the sample with dry KBr powder (typically 1-2% sample by weight).
- Compressing the mixture under high pressure in a pellet press to form a transparent disc.
Advantages and Disadvantages
The KBr pellet technique is versatile and can be applied to a wide range of solid samples. However, the technique requires careful preparation to avoid moisture contamination, which can lead to broad O-H absorption bands in the spectrum. Moreover, incomplete grinding can result in scattering effects.
Attenuated Total Reflectance (ATR): A Versatile Technique
Attenuated Total Reflectance (ATR) is a surface-sensitive technique that requires minimal or no sample preparation. The sample is placed in contact with an ATR crystal (e.g., diamond, germanium, zinc selenide), and the IR beam is directed through the crystal at an angle that causes total internal reflection.
Principles of ATR
At each reflection point, an evanescent wave penetrates a short distance into the sample. The depth of penetration is typically on the order of a few micrometers. The evanescent wave interacts with the sample, and the reflected beam carries information about the sample's IR absorption properties.
Applications
ATR is particularly useful for analyzing:
- Liquids.
- Pastes.
- Powders.
- Films.
- Surfaces.
It circumvents the need for extensive sample preparation, and it can be applied to strongly absorbing materials that are difficult to analyze using transmission techniques.
Advantages and Disadvantages
ATR offers the advantages of simplicity, minimal sample preparation, and the ability to analyze a wide range of sample types. However, ATR spectra may differ slightly from transmission spectra due to refractive index effects, and the technique is sensitive to the quality of contact between the sample and the ATR crystal.
Spectral Interpretation of Isopentyl Acetate: Identifying Key Absorption Bands
[Sample Preparation Techniques for IR Spectroscopy: Optimizing Your Analysis Understanding Wavenumber and Transmittance/Absorbance: Key Spectral Parameters transitions us to the instrumentation that measures these parameters. However, even with the best instrumentation, poor sample preparation can undermine the accuracy and reliability of IR spectroscopic analysis. Therefore, a thorough understanding of spectral interpretation is paramount.]
This section delves into the nuanced art of deciphering the IR spectrum of isopentyl acetate, focusing on the correlation between its distinctive molecular structure and the resulting absorption bands. Accurate band identification is crucial for confirming the presence and assessing the purity of this ester, prized for its banana-like aroma.
Carbonyl Group (C=O) Absorption
The carbonyl group (C=O) represents one of the most prominent and readily identifiable features in the IR spectrum of isopentyl acetate.
This strong absorption typically appears within the range of 1735-1750 cm-1.
Its high intensity arises from the significant dipole moment associated with the carbonyl bond.
The precise position of this band can be influenced by factors such as the electronic environment and any neighboring functional groups within the molecule.
A sharp, intense peak in this region serves as a definitive indicator of the ester functionality in isopentyl acetate.
C-H Bond Absorptions
Isopentyl acetate contains a variety of C-H bonds, each contributing to the spectrum with characteristic absorptions.
Alkyl C-H Stretching
These absorptions are generally observed in the region of 2850-3000 cm-1.
Symmetric and asymmetric stretches of methyl (-CH3) and methylene (-CH2-) groups contribute to the complexity of this region.
The intensity of these bands is proportional to the number of C-H bonds present.
C-H Bending
In-plane bending vibrations (scissoring) for -CH2- groups occur around 1465 cm-1.
Methyl group (-CH3) bending modes are typically found near 1375 cm-1.
These bending modes are useful for confirming the presence of alkyl groups within the isopentyl acetate molecule.
C-O Bond Absorptions
The ester linkage (C-O) in isopentyl acetate gives rise to characteristic absorptions in the 1000-1300 cm-1 region.
These absorptions are due to the stretching vibrations of the C-O bonds.
Two distinct bands are typically observed, corresponding to the C-O single bonds adjacent to the carbonyl group and the alkyl chain, respectively.
The band near 1240 cm-1 is commonly attributed to the C-O stretch adjacent to the carbonyl, whereas the band near 1050 cm-1 is assigned to the C-O stretch linked to the isopentyl group.
These bands, while less intense than the carbonyl absorption, are critical for confirming the presence of the ester functionality.
Acetate Group Characteristics
The acetate group (CH3COO-) as a whole exhibits specific spectral features.
The methyl group attached to the carbonyl carbon shows a C-H stretch around 3000cm-1
A methyl C-H bending vibration can be observed near 1375 cm-1.
This bending vibration in combination with C-O stretch vibration confirms the existence of the Acetate group.
By carefully analyzing the positions and intensities of these characteristic absorption bands, a detailed understanding of the molecular structure and purity of isopentyl acetate can be obtained. Spectral interpretation is thus an essential tool in the analytical chemist's arsenal.
Identifying Impurities in Isopentyl Acetate: A Spectroscopic Detective
Spectral Interpretation of Isopentyl Acetate: Identifying Key Absorption Bands and the considerations of optimising our analysis through Sample Preparation Techniques transitions us to the role of IR as a detective, sniffing out unwanted components. However, even with the best instrumentation and careful sample preparation, the presence of impurities can complicate the interpretation of IR spectra. Identifying these impurities is crucial for assessing the quality and suitability of isopentyl acetate for its intended application. This requires a keen understanding of potential contaminants and their characteristic spectral signatures.
The Silent Invaders: Common Impurities in Isopentyl Acetate
Isopentyl acetate, while widely used, is susceptible to contamination from various sources. Water is a frequent culprit, introduced during synthesis, storage, or handling. Other potential contaminants include residual solvents used in the production process (e.g., ethanol, ethyl acetate), unreacted starting materials (e.g., isopentyl alcohol, acetic acid), or degradation products formed during storage (e.g., isopentyl alcohol, acetic acid from ester hydrolysis).
Water: The Ubiquitous Contaminant
Water is arguably the most common impurity encountered in isopentyl acetate. Its presence is readily detectable in the IR spectrum due to its distinctive absorption bands.
Identifying O-H Stretches
The most prominent feature is the broad O-H stretching band, which typically appears in the region of 3600-3200 cm-1. This broadness is due to hydrogen bonding between water molecules. The intensity of this band is directly proportional to the concentration of water present. Even trace amounts of water can produce a noticeable signal.
Analyzing Bending Modes
Additionally, water exhibits a bending mode around 1640 cm-1. Although often less intense than the stretching band, it can provide confirmatory evidence. Careful examination of both the stretching and bending regions is crucial for accurate water detection.
Spotting other Culprits: Detecting Common Organic Impurities
Beyond water, several organic impurities can infiltrate isopentyl acetate. Identifying these requires a careful comparison of the sample spectrum with reference spectra of potential contaminants.
Residual Solvents
If ethanol or ethyl acetate is used in the synthesis or processing of isopentyl acetate, residual amounts may remain. Ethanol will exhibit a characteristic C-O stretch around 1050 cm-1, and ethyl acetate will show a distinctive ester carbonyl peak at a slightly different wavenumber than isopentyl acetate, along with other fingerprint region variations.
Unreacted Starting Materials
Unreacted isopentyl alcohol displays a sharp O-H stretch around 3650 cm-1, which is narrower and higher in wavenumber compared to the broad O-H stretch of water. Acetic acid, if present, will contribute a carbonyl peak as well, but its acidic O-H stretch will be broader and shifted to lower wavenumbers.
Degradation Products
Hydrolysis of isopentyl acetate produces isopentyl alcohol and acetic acid. Detecting these degradation products can indicate the age or improper storage of the sample. Monitoring the increase in the alcohol and acid peaks over time can be a useful indicator of sample degradation.
The Spectroscopic Detective's Toolkit: Best Practices
Successfully identifying impurities in isopentyl acetate requires a systematic approach. Begin by obtaining a high-quality IR spectrum of the sample. Compare this spectrum to a reference spectrum of pure isopentyl acetate.
Pay close attention to any additional peaks or shoulders that are not present in the reference spectrum. Consult spectral databases and reference materials to identify potential contaminants based on the location and shape of these peaks.
Finally, consider the sample's history and potential sources of contamination when interpreting the results. With careful analysis and a thorough understanding of IR spectroscopy, you can effectively identify and quantify impurities in isopentyl acetate.
The Fingerprint Region: Unlocking Unique Molecular Signatures
Identifying Impurities in Isopentyl Acetate: A Spectroscopic Detective, Spectral Interpretation of Isopentyl Acetate: Identifying Key Absorption Bands and the considerations of optimising our analysis through Sample Preparation Techniques transitions us to the role of IR as a detective, sniffing out unwanted components. However, even with the best interpretation of characteristic group frequencies, differentiation between structurally similar molecules can be challenging. This is where the fingerprint region of the IR spectrum becomes invaluable.
Deciphering Molecular Identity: The Fingerprint Region Explained
The fingerprint region, typically spanning from approximately 1500 cm⁻¹ to 500 cm⁻¹, is a complex area of the IR spectrum characterized by numerous overlapping absorption bands. These bands arise from a variety of vibrational modes, including:
- Skeletal vibrations.
- C-O-H bending modes.
- C-C stretching and bending modes.
The complexity arises because these vibrations are highly sensitive to the entire molecular structure, including subtle differences in conformation and neighboring groups.
This makes the fingerprint region unique for each compound, acting as a distinctive "fingerprint" that allows for definitive identification.
Distinguishing the Indistinguishable: The Power of Unique Signatures
While characteristic group frequencies can point to the presence of certain functional groups, they often lack the specificity required to differentiate between closely related compounds.
For instance, distinguishing between isomers or compounds with similar functional groups can be extremely difficult based solely on the functional group region (above 1500 cm⁻¹).
The fingerprint region provides a level of detail that is simply not available elsewhere in the spectrum. Small changes in molecular structure result in significant alterations in the fingerprint region, allowing for confident differentiation between compounds that might otherwise appear identical.
Spectral Libraries: Your Guide to Molecular Identification
The true power of the fingerprint region is unleashed when used in conjunction with spectral libraries. These libraries contain a vast collection of reference IR spectra for a wide range of compounds.
By comparing the fingerprint region of an unknown sample with the spectra in a library, it is possible to identify the compound with a high degree of confidence.
Leveraging Spectral Libraries for Compound Matching
The process involves:
-
Acquiring a high-quality IR spectrum of the unknown sample.
-
Focusing on the fingerprint region (1500 cm⁻¹ to 500 cm⁻¹).
-
Using specialized software to compare the unknown spectrum with the spectra in the library.
The software typically employs algorithms that search for the best match based on peak positions, intensities, and overall spectral shape.
The resulting match provides a probable identification of the unknown compound, which can then be further validated using other analytical techniques, or chemical intuition.
Considerations for Accurate Library Matching
It is crucial to ensure that the reference spectra in the library were obtained under similar conditions as the unknown sample. Factors such as:
- Instrument type.
- Resolution.
- Sample preparation method.
can all influence the appearance of the spectrum. Using libraries with well-documented experimental parameters will significantly improve the accuracy of compound identification.
Spectroscopic Data Analysis: Tools and Techniques for Interpretation
The Fingerprint Region: Unlocking Unique Molecular Signatures, Identifying Impurities in Isopentyl Acetate: A Spectroscopic Detective, Spectral Interpretation of Isopentyl Acetate: Identifying Key Absorption Bands and the considerations of optimising our analysis through Sample Preparation Techniques transitions us to the role of IR as a detective, where the acquired spectral data serves as crucial evidence. The subsequent interpretation of this evidence then becomes paramount. To fully harness the power of IR spectroscopy, a robust understanding of the tools and techniques employed in spectroscopic data analysis is necessary. This section will guide you through these techniques, with a specific focus on qualitative analysis for definitively identifying the presence of isopentyl acetate.
Essential Techniques for Spectral Interpretation
Spectral interpretation is not a passive process; it requires active engagement with the data and a systematic approach. Several key techniques facilitate this process, allowing for accurate identification and characterization of compounds.
Peak Identification: Locating and Assigning Absorption Bands
The first crucial step in spectral interpretation is peak identification. This involves precisely locating the absorption bands within the IR spectrum and determining their corresponding wavenumbers. Accurate wavenumber determination is critical because it allows for the association of specific peaks with vibrational modes of particular functional groups.
Software tools often assist in this process, providing automated peak-picking functions. However, a trained spectroscopist must always critically evaluate these automated results to ensure accuracy and to distinguish genuine peaks from noise or artifacts.
Intensity Analysis: Unveiling Molecular Quantities
The intensity of an absorption band is directly proportional to the change in dipole moment during vibration, as well as the concentration of the absorbing species. Stronger absorption bands indicate a greater change in dipole moment or a higher concentration of the corresponding functional group.
Careful analysis of peak intensities can provide insights into the relative abundance of different functional groups within the sample. Moreover, when coupled with calibration curves, intensity measurements can be utilized for quantitative analysis, thus to determine the exact amount of the target compound.
Band Shape Analysis: Gaining Molecular Insights
The shape of an IR absorption band can also provide valuable information about the sample. Broad bands may indicate the presence of hydrogen bonding, while sharp, well-defined bands typically suggest a more ordered environment.
Careful examination of band shapes can help distinguish between different isomers or conformational states of a molecule. Furthermore, band shapes can be affected by factors such as temperature and solvent, providing insights into intermolecular interactions.
Qualitative Analysis: Confirming the Presence of Isopentyl Acetate
Qualitative analysis in IR spectroscopy aims to confirm the presence of a specific compound within a sample. For isopentyl acetate, this involves comparing the acquired IR spectrum to a reference spectrum and identifying characteristic absorption bands.
Matching Key Absorption Bands
The most direct approach to qualitative analysis is to compare the acquired spectrum to a reference spectrum of pure isopentyl acetate. If the sample contains isopentyl acetate, the key absorption bands corresponding to its functional groups (carbonyl, C-H, C-O) should be present at their characteristic wavenumbers.
However, it is critical to consider the possibility of spectral shifts due to matrix effects or intermolecular interactions. A close match in both peak position and relative intensity is essential for a confident identification.
The Role of Spectral Libraries
Spectral libraries, such as those available from NIST and SDBS, can be invaluable resources for qualitative analysis. These libraries contain reference spectra for a vast array of compounds, including isopentyl acetate.
By comparing the unknown spectrum to the library spectra, one can quickly assess the likelihood of isopentyl acetate being present. Automated spectral matching algorithms can further assist in this process, but manual evaluation by a trained spectroscopist remains crucial.
Ruling Out Interferences
Qualitative analysis is not just about confirming the presence of a target compound. It also involves ruling out potential interferences from other components in the sample. Identifying and accounting for the absorption bands of known impurities is an essential step in ensuring an accurate identification of isopentyl acetate.
Applications of IR Spectroscopy in Isopentyl Acetate Analysis: Beyond Identification
Having established the fundamentals of IR spectroscopy and its utility in identifying isopentyl acetate, it's important to move beyond simple identification and explore the technique's broader applications. IR spectroscopy offers a powerful toolkit for quality control, reaction monitoring, and quantitative analysis, providing valuable insights into the properties and behavior of this important ester.
Quality Control and Assurance
IR spectroscopy is invaluable in quality control, ensuring the identity and purity of isopentyl acetate in various applications.
The technique allows for rapid and non-destructive verification of the compound's authenticity, confirming that the received material matches the expected spectral fingerprint.
By comparing the sample's IR spectrum to a reference standard, any deviations can be quickly identified, indicating potential contamination or degradation.
This comparative analysis is crucial in industries where consistent quality is paramount, such as in the flavor and fragrance sectors.
Purity Assessment
Beyond simple identification, IR spectroscopy can also be used to assess the purity of isopentyl acetate.
The presence of extraneous peaks in the spectrum, indicative of impurities, can be readily detected.
The relative intensity of these peaks can provide a semi-quantitative estimate of the impurity levels.
Furthermore, the absence of specific peaks associated with common contaminants, such as water or residual solvents, can provide reassurance regarding the compound's purity.
Reaction Monitoring
IR spectroscopy offers a unique advantage in monitoring chemical reactions involving isopentyl acetate.
By tracking the appearance or disappearance of characteristic absorption bands, the progress of the reaction can be followed in real-time.
This capability is particularly useful in optimizing reaction conditions and understanding reaction kinetics.
In-Situ Monitoring
One powerful application is in-situ monitoring, where the IR spectrometer is directly coupled to the reaction vessel.
This allows for continuous monitoring of the reaction mixture without the need for sample extraction or preparation.
Changes in the concentrations of reactants and products can be tracked by observing the corresponding changes in the intensities of their IR absorption bands.
This provides valuable information about the reaction rate, endpoint, and selectivity.
Quantitative Analysis
IR spectroscopy can be employed for the quantitative determination of isopentyl acetate concentration in a sample.
This relies on the Beer-Lambert Law, which states that the absorbance of a substance is directly proportional to its concentration and path length.
By measuring the absorbance of a characteristic absorption band at a specific wavenumber, the concentration of isopentyl acetate can be calculated.
Calibration Curves
For accurate quantitative analysis, a calibration curve must be generated.
This involves measuring the absorbance of a series of standard solutions of known concentrations and plotting the absorbance against the concentration.
The resulting calibration curve can then be used to determine the concentration of isopentyl acetate in unknown samples.
Careful attention must be paid to factors such as baseline correction, peak selection, and instrument calibration to ensure accurate and reliable results.
Multivariate Analysis
In complex mixtures, where overlapping absorption bands may complicate quantitative analysis, multivariate analysis techniques can be employed.
These techniques use sophisticated algorithms to deconvolve the overlapping peaks and accurately determine the concentration of each component.
This is particularly useful in analyzing complex flavor formulations or reaction mixtures containing multiple products.
Spectral Resources and Databases: Expanding Your Knowledge
Having established the fundamentals of IR spectroscopy and its utility in identifying isopentyl acetate, it's important to move beyond simple identification and explore the technique's broader applications. IR spectroscopy offers a powerful toolkit for quality control, reaction monitoring, and advanced analysis. To fully leverage its potential, a deep understanding of available spectral resources and databases is essential.
These resources provide the crucial reference points and analytical tools needed to extract meaningful insights from IR spectra. They allow researchers and analysts to go beyond basic identification and delve into the intricacies of molecular structure and composition.
Spectral Libraries: The Foundation of Compound Identification
Spectral libraries are collections of reference spectra of known compounds. These libraries serve as the cornerstone for identifying unknown substances by comparing their spectra to the database entries. Spectral matching algorithms then quantify the degree of overlap between the query spectrum and each library entry.
Several types of spectral libraries are available, differing in their scope and quality. Commercial libraries typically offer high-quality spectra meticulously curated and validated. Open-source libraries, while generally less curated, can be a valuable supplement, especially for commonly encountered compounds.
Effective use of spectral libraries hinges on understanding their limitations. Factors such as spectral resolution, sample preparation, and instrument calibration can influence the quality of the acquired spectrum. Consequently, careful consideration must be given to these factors to ensure accurate spectral matching.
Software Tools for Spectral Analysis: Enhancing Data Interpretation
Software tools play a crucial role in processing and interpreting IR spectra. These tools often incorporate advanced algorithms for baseline correction, peak fitting, and spectral deconvolution.
Baseline correction removes unwanted background signals, improving the accuracy of peak identification and quantification. Peak fitting allows for the precise determination of peak positions and intensities, even in complex spectra with overlapping bands.
Furthermore, specialized software packages offer features such as spectral searching, library building, and chemometric analysis. These tools empower users to extract detailed information from IR spectra, facilitating a deeper understanding of molecular composition and dynamics.
Navigating the NIST Chemistry WebBook: A Comprehensive Online Resource
The NIST Chemistry WebBook, maintained by the National Institute of Standards and Technology (NIST), is an invaluable online resource for chemists. It provides access to a vast repository of chemical and physical property data, including IR spectra for thousands of compounds.
To utilize the WebBook, one can search for isopentyl acetate or other compounds of interest by name, CAS registry number, or molecular formula. The database provides access to reference spectra, along with other relevant data such as thermodynamic properties, ionization energies, and vibrational frequencies.
The IR spectra available in the NIST Chemistry WebBook are typically acquired under carefully controlled conditions, making them a reliable reference for spectral matching and compound identification.
Exploring the SDBS Database: A Public Spectral Repository
The Spectral Database for Organic Compounds (SDBS), maintained by the National Institute of Advanced Industrial Science and Technology (AIST) in Japan, is another significant public resource. It contains a large collection of IR spectra, along with data from other spectroscopic techniques such as NMR and mass spectrometry.
SDBS offers a user-friendly interface for searching and browsing spectra. Users can search by compound name, molecular formula, or CAS registry number. The database provides access to both experimental spectra and simulated spectra, which can be helpful for understanding complex spectral features.
While SDBS is a valuable resource, it's important to note that the quality and accuracy of the spectra may vary. Users should critically evaluate the data and compare it with other sources when possible.
By leveraging these spectral resources and databases, researchers and analysts can unlock the full potential of IR spectroscopy for the comprehensive analysis of isopentyl acetate and other chemical compounds.
Video: Isopentyl Acetate IR: Spectroscopy Guide
FAQs: Isopentyl Acetate IR Spectroscopy
What are the key peaks to look for when identifying isopentyl acetate ir spectra?
Key peaks for identifying isopentyl acetate ir are typically found around 1740 cm-1 (C=O stretch of the ester), 1240 cm-1 (C-O stretch), and a cluster of peaks between 2800-3000 cm-1 (C-H stretches). These, along with other signals, confirm the presence of functional groups characteristic of isopentyl acetate.
Why is the carbonyl (C=O) stretch important in isopentyl acetate ir spectroscopy?
The strong carbonyl (C=O) stretch around 1740 cm-1 is crucial because it is a very intense and characteristic peak for esters. This peak in the isopentyl acetate ir spectrum strongly indicates the presence of the ester functional group, a key part of its molecular structure.
What other compounds might have similar IR spectra to isopentyl acetate, and how can I differentiate them?
Other esters could show similar IR spectra. Differentiating them requires careful consideration of the entire spectrum, especially the fingerprint region (below 1500 cm-1) where unique bending and stretching vibrations occur. Comparing against known spectra of other esters helps identify the isopentyl acetate ir spectrum specifically.
How can you use IR spectroscopy to determine the purity of isopentyl acetate?
By comparing the intensities of characteristic isopentyl acetate ir peaks to peaks of known impurities, the relative amount of each compound can be estimated. The absence of peaks associated with unwanted byproducts, or unusually intense peaks, provide an indication of isopentyl acetate purity.
So, there you have it! Hopefully, this guide gives you a better handle on interpreting Isopentyl Acetate IR spectra. Remember, practice makes perfect, so get in the lab and start comparing your own spectra to the information we've covered. Happy analyzing!