Hydrogen's Hidden States: Decoding the Phase Diagram
The study of high-pressure physics is crucial for understanding the phase diagram of hydrogen. Scientists at the Lawrence Livermore National Laboratory contribute significantly to our knowledge of hydrogen's behavior under extreme conditions. Sophisticated diamond anvil cells allow researchers to explore these exotic states of matter. Predictions from computational methods developed by Isaac Silvera, for instance, provide valuable theoretical insights for interpreting experimental data concerning the elusive phase diagram of hydrogen.
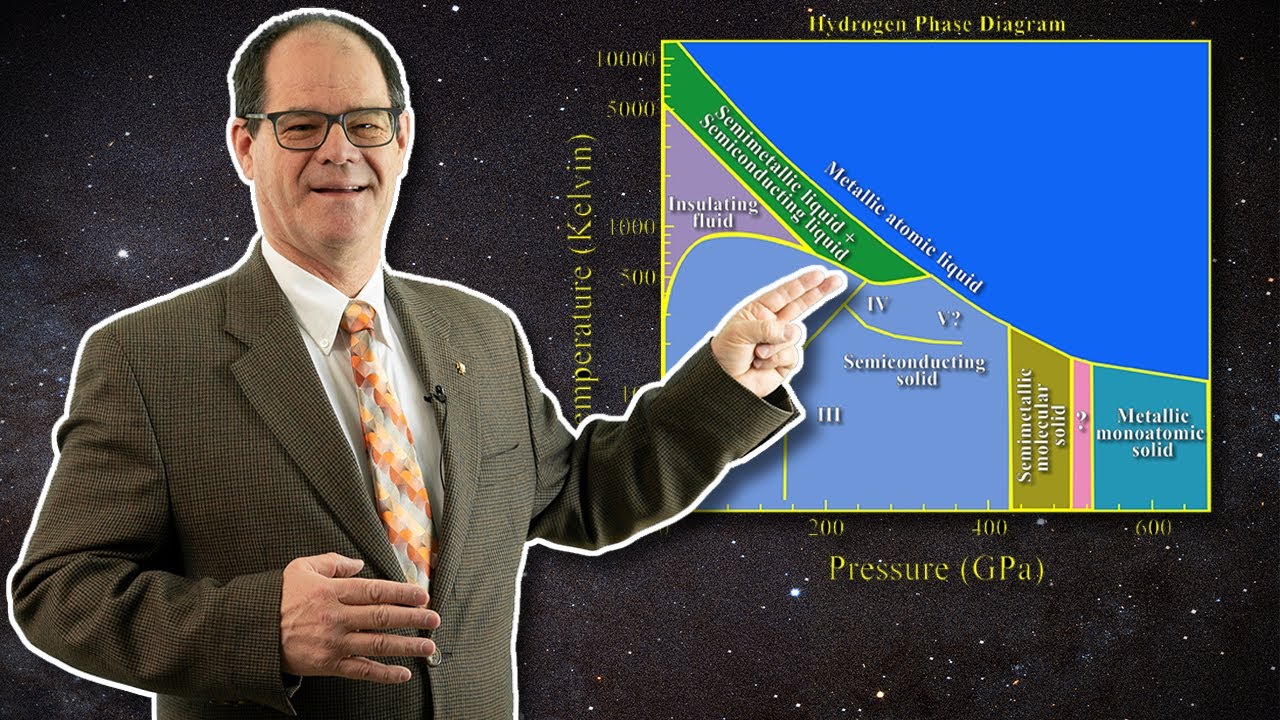
Image taken from the YouTube channel Sky Scholar , from the video titled The Hydrogen Phase Diagram & Evidence for the Liquid Metallic Hydrogen Solar Model! .
Hydrogen, the simplest and most abundant element in the universe, belies its apparent simplicity with a remarkably complex phase diagram. Its behavior under extreme conditions of temperature and pressure continues to challenge our understanding of matter. This pursuit of knowledge about its varied phases has captivated scientists for decades.
Hydrogen: More Than Just a Simple Element
Hydrogen's fundamental nature as a single proton and electron system makes it a cornerstone of chemistry and physics. Its role in stellar processes, as a primary component of gas giants, and as a potential energy source on Earth, underscores its significance. However, the extreme conditions required to probe its exotic phases, such as metallic hydrogen, make it a particularly difficult subject of study.
Decoding the Roadmap: Understanding Phase Diagrams
A phase diagram serves as a roadmap that maps out the different states of matter an element can exist in. These diagrams plot temperature against pressure, outlining the conditions under which different phases—solid, liquid, gas, and other more exotic states—are stable. Understanding a substance's phase diagram is crucial for predicting its behavior in various environments.
For hydrogen, mapping this diagram, especially at extreme pressures, is a complex and ongoing endeavor. The location and nature of phase boundaries are still actively researched.
The Allure of Extreme Conditions: Why Hydrogen Captivates Scientists
The extreme conditions needed to explore the high-pressure phases of hydrogen push the boundaries of experimental and theoretical capabilities. Creating and maintaining these conditions requires specialized equipment and sophisticated computational techniques.
The potential discovery of novel phases, such as metallic hydrogen with its predicted superconducting properties, continues to fuel scientific interest. Moreover, a deeper understanding of hydrogen's phase diagram is vital for modeling the interiors of giant planets like Jupiter and Saturn. This makes it a critical area of research with far-reaching implications across astrophysics and condensed matter physics.
Fundamentals: Delving into Hydrogen and Phase Diagrams
Understanding hydrogen's exotic phases necessitates a firm grasp of its fundamental nature and the tools we use to map its behavior. Hydrogen's deceptively simple atomic structure gives rise to unusual properties, especially under extreme conditions. Concurrently, understanding phase diagrams, particularly their axes of temperature and pressure, is critical to predicting hydrogen's state in various environments.
The Peculiar Nature of Hydrogen
Hydrogen stands out due to its small atomic size, making it the simplest and most abundant element in the universe. Its behavior, however, is far from simple, especially when subjected to extreme pressures.
The small mass of the hydrogen atom leads to significant quantum effects, influencing its behavior at low temperatures and high densities. These effects are far more pronounced in hydrogen than in heavier elements.
Zero-point energy, a consequence of quantum mechanics, plays a crucial role in determining the stability of different phases. It manifests itself as the minimum energy a quantum mechanical system possesses, even at absolute zero temperature. This energy significantly affects hydrogen's solid and liquid phases.
Decoding Phase Diagrams
A phase diagram is essentially a map showing the stable states of a substance under different conditions. It is a graphical representation of the physical states of a substance under varying conditions of temperature and pressure. These diagrams are essential for predicting how a material will behave in different environments.
These diagrams plot pressure (typically in Pascals or Gigapascals) on one axis and temperature (in Kelvin or Celsius) on the other. The lines on the diagram, known as phase boundaries, indicate the conditions under which two or more phases can coexist in equilibrium.
Common Phases of Hydrogen
The most familiar phases are gas, liquid, and solid.
Gaseous hydrogen is the state most commonly encountered at ambient temperatures and pressures. Liquid hydrogen is formed at cryogenic temperatures, while solid hydrogen forms under increased pressure.
Under extreme pressures, hydrogen is predicted to transition into a metallic phase. This metallic phase is characterized by high electrical conductivity, similar to that of metals.
The Role of Temperature and Pressure
Temperature and pressure are the key variables that determine the phase of a substance.
Temperature governs the kinetic energy of atoms or molecules. Higher temperatures favor phases with greater disorder, such as gases.
Pressure, on the other hand, forces atoms or molecules closer together, favoring denser phases like liquids and solids. Under extreme pressure, the electronic structure of atoms can be altered, leading to phase transitions.
In the case of hydrogen, the interplay between temperature and pressure is particularly complex due to the strong influence of quantum effects.
Experimental Challenges
Mapping the phase diagram of hydrogen, particularly at high pressures, is a formidable experimental challenge. Creating and maintaining the extreme conditions needed to probe these exotic phases requires specialized equipment, such as diamond anvil cells.
Reaching pressures of hundreds of Gigapascals and maintaining precise temperature control simultaneously is technically demanding. Moreover, accurately measuring the properties of hydrogen under these conditions is difficult, adding further complexity to the problem.
Familiar Territory: Exploring the Common States of Hydrogen
Having established the fundamental properties of hydrogen and the significance of phase diagrams in predicting its behavior, let's now turn our attention to the familiar forms in which we encounter this ubiquitous element. While the exotic phases of hydrogen under extreme pressures capture much of the scientific attention, understanding its more common gaseous, liquid, and solid states is crucial for appreciating its versatility and potential.
Gaseous Hydrogen: Properties and Applications
Under standard conditions, hydrogen exists as a colorless, odorless, and tasteless gas. Its low density, being the lightest gas, gives it unique buoyancy properties. This has historically led to its use in airships and balloons, though flammability concerns have largely superseded this application.
The molecule exists as H₂, two hydrogen atoms covalently bonded. The gas is relatively inert at room temperature, but readily participates in chemical reactions when heated or with the aid of catalysts. This reactivity makes it an invaluable industrial feedstock.
Gaseous hydrogen plays a vital role in the Haber-Bosch process for ammonia production, a cornerstone of modern agriculture. It is also used extensively in the hydrogenation of vegetable oils, converting them into solid or semi-solid fats used in food products. The petroleum industry relies heavily on hydrogen for hydrocracking and hydrodesulfurization processes, essential for refining crude oil and removing sulfur contaminants.
Fuel cells represent a promising application, converting chemical energy into electricity with water as the only byproduct. Hydrogen's high energy content makes it an attractive fuel, but challenges related to storage, transportation, and infrastructure hinder widespread adoption.
Liquid Hydrogen: Cryogenic Challenges and Rocket Fuel
To transform hydrogen into its liquid state, it must be cooled to extremely low temperatures (around -253°C or 20 K). This cryogenic requirement presents significant engineering challenges, demanding specialized storage tanks with exceptional insulation to minimize boil-off.
The energy density of liquid hydrogen, while high on a mass basis, is lower on a volumetric basis compared to conventional fuels. This means larger tanks are needed to store a given amount of energy.
Despite these challenges, liquid hydrogen finds a crucial application as a rocket propellant. Its high specific impulse – a measure of the thrust produced per unit of propellant consumed – makes it ideal for propelling rockets and spacecraft.
The combination of liquid hydrogen and liquid oxygen provides one of the most efficient chemical propulsion systems currently available, powering the main engines of the Space Shuttle and many other launch vehicles.
Solid Hydrogen: Formation and Molecular Structure
Solid hydrogen forms when liquid hydrogen is further cooled to its freezing point, reaching temperatures near absolute zero. Under moderate pressures, solid hydrogen adopts a molecular structure, retaining the H₂ diatomic form. The molecules are arranged in a crystalline lattice.
The weak intermolecular forces between hydrogen molecules in the solid state result in a relatively soft and compressible material. Increasing pressure causes the molecules to pack more closely together, eventually leading to changes in the crystal structure.
Research into solid hydrogen focuses on understanding its behavior at high densities and low temperatures, conditions where quantum effects become prominent. The arrangement of the molecules, their rotational and vibrational states, and the nature of the intermolecular interactions are all subjects of intense investigation.
Understanding solid hydrogen is vital to understanding the properties of larger gas giants. The behavior under these conditions offers important insights into the more complex phase diagram at extreme pressures.
The Holy Grail: The Quest for Metallic Hydrogen
Having explored the familiar gaseous, liquid, and solid states of hydrogen, we now venture into more speculative territory: the pursuit of metallic hydrogen. This exotic phase, theorized nearly a century ago, has become a "holy grail" for physicists and materials scientists alike, promising revolutionary advancements if synthesized and stabilized. The extreme conditions required to achieve this transformation, coupled with the potential for groundbreaking technological applications, fuel the ongoing scientific endeavor.
The Theoretical Foundation: Wigner, Huntington, and the Metallic Promise
The journey towards metallic hydrogen began in 1935 with a groundbreaking prediction by Eugene Wigner and H.B. Huntington. Their theoretical calculations suggested that under immense pressure, hydrogen, normally an insulator, would undergo a phase transition to a metallic state.
In this metallic phase, the electrons, normally bound to individual hydrogen atoms, would become delocalized, freely conducting electricity much like in conventional metals. This Wigner-Huntington transition represented a radical shift in our understanding of matter under extreme conditions.
The implications of metallic hydrogen are far-reaching. Theoretical calculations suggest that it could be a room-temperature superconductor, a material that conducts electricity with zero resistance at ambient temperatures. Such a material would revolutionize energy transmission, storage, and countless other technologies.
High Pressure: The Key to Metallization
The transformation of hydrogen into a metallic state hinges on the application of immense pressure. Pressure forces the hydrogen molecules closer together, compressing the electron clouds and eventually leading to the delocalization of electrons necessary for metallic conductivity.
The pressures required are staggering, initially predicted to be around 25 GPa, but later refined to several hundred GPa. These pressures are far beyond what can be achieved with conventional laboratory equipment.
This need for extreme compression has driven the development of specialized high-pressure techniques, pushing the boundaries of materials science and engineering.
The Diamond Anvil Cell: A Window into Extreme Conditions
The diamond anvil cell (DAC) has become the indispensable tool in the quest for metallic hydrogen. This ingenious device uses two opposing diamonds to squeeze a tiny sample of hydrogen to extreme pressures.
Diamonds are exceptionally hard and transparent, allowing researchers to both compress the sample and observe its behavior using various spectroscopic techniques.
The DAC has revolutionized high-pressure research, enabling scientists to probe the properties of matter under conditions previously unimaginable.
However, working with DACs at these pressures is fraught with challenges. Maintaining stable pressure, preventing hydrogen from leaking or reacting with the diamond, and accurately measuring the pressure are all significant hurdles.
Experimental Efforts and Enduring Challenges
Despite the theoretical predictions and technological advancements, the synthesis of metallic hydrogen remains an ongoing challenge.
Numerous research groups have reported tantalizing hints of metallization, but definitive proof has been elusive. Experimental difficulties, such as accurately characterizing the sample under extreme conditions, have led to controversies and debates within the scientific community.
Neil Ashcroft, a prominent figure in condensed matter physics, made significant contributions to the theoretical understanding of metallic hydrogen and its potential for superconductivity. His work has inspired and guided numerous experimental efforts.
Researchers face a multitude of challenges, from sample preparation and containment to accurate pressure measurement and the interpretation of experimental data. The extreme conditions often lead to unexpected phenomena, requiring careful analysis and validation. The quest continues, driven by the tantalizing promise of a revolutionary material that could reshape our technological landscape.
Having pushed hydrogen to the limits of experimental capabilities, the challenge now lies in interpreting the intricate dance of atoms and electrons within. Deciphering the complex phase diagram of hydrogen requires a deep dive into the quantum realm and sophisticated theoretical tools, acknowledging that the map we're drawing is still incomplete.
Decoding the Puzzle: Understanding the Complex Phase Diagram
The behavior of hydrogen under extreme conditions defies simple classical models. Its lightness and the resulting quantum effects play a pivotal role in shaping its phase diagram. These effects, often negligible in heavier elements, become dominant in hydrogen, introducing complexities that demand advanced theoretical treatment.
The Quantum Influence
Hydrogen's phase transitions are heavily influenced by quantum effects. The uncertainty principle dictates that the position and momentum of hydrogen atoms cannot be simultaneously known with perfect accuracy.
This leads to a significant zero-point energy, a minimum energy that the atoms possess even at absolute zero temperature. This zero-point energy acts as an effective pressure, resisting the compression induced by external forces.
It affects the stability of different phases and alters the transition pressures between them. Consequently, classical simulations, which neglect these quantum fluctuations, often fail to accurately predict the phase diagram of hydrogen.
Equations of State: Mapping the Thermodynamic Landscape
Equations of state (EOS) serve as crucial bridges between macroscopic properties like pressure, volume, and temperature, and the microscopic behavior of hydrogen. They are mathematical relationships that define the state of a substance under specific conditions.
Accurate EOS are essential for predicting phase boundaries and understanding the thermodynamic stability of different hydrogen phases.
Various EOS models exist, ranging from simple analytical approximations to complex numerical simulations. Each model has its limitations, particularly at extreme pressures and temperatures, where the underlying assumptions may break down.
The quest for a universally accurate EOS for hydrogen remains a central challenge in the field.
Density Functional Theory (DFT): A Quantum Mechanical Microscope
Density Functional Theory (DFT) has emerged as a powerful tool for probing the electronic structure of hydrogen and predicting its phase diagram. DFT is a quantum mechanical method that calculates the electronic properties of a system based on its electron density.
It allows researchers to simulate the behavior of hydrogen atoms and electrons under extreme pressures, providing valuable insights into phase transitions and material properties.
DFT calculations can predict the crystal structures of solid hydrogen, the metallization pressure, and even the potential for superconductivity. First-principles calculations, based on DFT, are particularly valuable as they rely on fundamental physical laws without empirical parameters.
However, DFT is not without its limitations. Approximations within DFT, such as the exchange-correlation functional, can significantly affect the accuracy of the results.
Careful validation and benchmarking against experimental data are crucial for ensuring the reliability of DFT predictions.
Controversies and Uncertainties
Despite significant advances in theoretical modeling, the phase diagram of hydrogen remains a subject of debate. Discrepancies persist between different theoretical predictions and experimental observations, highlighting the challenges of accurately simulating matter under extreme conditions.
The precise nature of the metallic transition, the crystal structures of the different solid phases, and the existence of exotic phases like liquid metallic hydrogen are all areas of ongoing research and controversy.
These uncertainties underscore the need for continued refinement of theoretical models and experimental techniques.
Superconductivity: The Ultimate Prize?
One of the most tantalizing prospects associated with metallic hydrogen is the potential for room-temperature superconductivity. Theoretical calculations suggest that metallic hydrogen, particularly in certain crystal structures, could exhibit superconductivity at or near ambient temperatures.
This would revolutionize energy transmission and storage, enabling lossless electrical grids and ultra-efficient electronic devices. However, the experimental verification of superconductivity in metallic hydrogen remains elusive.
The high pressures required to synthesize the material and the challenges of measuring its electrical properties under such extreme conditions make this a formidable experimental task.
Having pushed hydrogen to the limits of experimental capabilities, the challenge now lies in interpreting the intricate dance of atoms and electrons within. Deciphering the complex phase diagram of hydrogen requires a deep dive into the quantum realm and sophisticated theoretical tools, acknowledging that the map we're drawing is still incomplete.
Advanced Computational Studies: Simulations and Predictions
The complexities inherent in hydrogen's behavior under extreme conditions demand more than just experimental observation. Advanced computational studies have become indispensable tools for simulating and predicting its phase transitions and properties. These simulations offer insights into regions of the phase diagram that are currently inaccessible to experiments, and provide a crucial bridge between theoretical models and experimental findings.
The Power of First-Principles Simulations
At the forefront of these computational endeavors are first-principles methods, most notably Density Functional Theory (DFT). DFT allows researchers to calculate the electronic structure of hydrogen systems, and to derive their ground-state properties with remarkable accuracy.
Unlike empirical methods that rely on experimental data, DFT operates on the fundamental laws of quantum mechanics, using only the atomic number as input. This "parameter-free" approach makes it particularly well-suited for exploring novel phases of hydrogen where experimental data is scarce or nonexistent.
However, DFT is not without its limitations. Approximations within DFT, particularly the exchange-correlation functional, can significantly affect the predicted transition pressures and the stability of different phases.
Molecular Dynamics: Simulating Atomic Motion
To study the dynamics of hydrogen atoms, researchers employ Molecular Dynamics (MD) simulations. MD involves solving Newton's equations of motion for a system of interacting atoms, allowing them to track the evolution of the system over time.
In the context of hydrogen, MD simulations can be used to investigate the melting behavior of solid phases, the diffusion of atoms in the liquid state, and the formation of molecular bonds under high pressure.
By combining DFT with MD, researchers can perform ab initio molecular dynamics (AIMD) simulations, where the forces on the atoms are calculated directly from electronic structure theory.
AIMD provides a highly accurate and detailed picture of the atomic-scale processes governing hydrogen's phase transitions.
Unveiling Phase Boundaries: Case Studies
Computational studies have played a crucial role in refining our understanding of hydrogen's phase diagram. Here are a few illustrative examples:
- The Liquid-Liquid Transition (LLT): Simulations have provided strong evidence for a LLT in dense hydrogen, where the liquid undergoes a transition from a molecular to an atomic state. These simulations predict a significant change in the electrical conductivity across the LLT, which has been partially confirmed by experiments.
- The Structure of Solid Phases: DFT calculations have been instrumental in identifying the stable crystal structures of solid hydrogen at different pressures. These calculations have revealed a complex sequence of structural transitions, involving the breaking and reforming of molecular bonds.
- Superconductivity: Theoretical predictions suggest that metallic hydrogen could be a high-temperature superconductor. Computational studies are being used to investigate the superconducting properties of different metallic phases, and to identify the factors that promote high-temperature superconductivity.
Visualizing Results: From Data to Insight
The output of these simulations is vast, often consisting of terabytes of data representing the positions and velocities of millions of atoms. Visualizing this data is crucial for extracting meaningful insights.
Researchers use a variety of techniques to visualize the results of their simulations, including:
- Snapshots of atomic configurations: These images provide a visual representation of the arrangement of atoms in a particular phase.
- Radial distribution functions: These functions quantify the probability of finding an atom at a certain distance from another atom, providing information about the local structure of the material.
- Phonon dispersion curves: These curves show the relationship between the frequency and wavelength of vibrational modes in the crystal lattice, providing insights into the stability and dynamic properties of the material.
Here's an example of the type of graph that is often used: Graph: A graph depicting pressure vs. temperature, showing a theoretical phase diagram of hydrogen with various solid, liquid, and metallic phases labeled. The graph should include lines representing phase boundaries, with error bars or shaded regions to indicate uncertainty. The axes should be clearly labeled with units. (A real visual representation of such a graph would be included here in an actual article.)
By combining advanced computational techniques with insightful visualizations, researchers are steadily unraveling the mysteries of hydrogen's phase diagram. These studies not only advance our fundamental understanding of matter under extreme conditions, but also have implications for planetary science and the search for novel materials with exotic properties.
Video: Hydrogen's Hidden States: Decoding the Phase Diagram
Decoding the Phase Diagram of Hydrogen: Frequently Asked Questions
The phase diagram of hydrogen can seem complex, so we've compiled some frequently asked questions to help you better understand its hidden states.
What are the key differences between the phases of hydrogen?
The different phases of hydrogen are determined by the arrangement and behavior of hydrogen atoms under various pressures and temperatures. Each phase exhibits unique properties, such as conductivity and structure. The phase diagram of hydrogen illustrates these transitions.
Why is understanding the phase diagram of hydrogen important?
Understanding the phase diagram of hydrogen is crucial for various applications, including energy storage, planetary science (understanding gas giants), and the development of new materials with novel properties. Knowing the stable phases at different conditions allows for controlled experimentation.
What is metallic hydrogen and why is it so sought after?
Metallic hydrogen is a phase of hydrogen where it becomes electrically conductive, similar to metals. It's highly sought after due to its potential for superconductivity at room temperature and its potential as a super-efficient energy storage material. Predicting its existence and properties relies on accurately mapping the phase diagram of hydrogen.
What challenges do scientists face when studying the phase diagram of hydrogen?
Studying the phase diagram of hydrogen presents significant challenges due to the extreme pressures and temperatures required to induce phase transitions. Experimental techniques capable of reaching these conditions are complex and often yield ambiguous results. This makes accurate theoretical modeling and experimental verification essential.