Kg of Hydrogen: How Much Do You Need?
Understanding the energy potential of hydrogen requires careful consideration of its physical properties, especially when evaluating its viability as a fuel source. The mass of hydrogen in kg directly influences storage requirements and transportation logistics, crucial factors for organizations such as the U.S. Department of Energy (DOE) that are heavily invested in hydrogen research. For instance, a standard hydrogen fuel cell vehicle necessitates a specific mass of hydrogen to achieve a targeted driving range, often measured using sophisticated analytical tools like gas chromatography-mass spectrometry (GC-MS) to ensure purity and accurate quantification. Furthermore, the National Renewable Energy Laboratory (NREL) conducts extensive research on optimizing hydrogen production and storage methods, where precise measurement of mass plays a pivotal role in assessing the efficiency and scalability of different approaches.
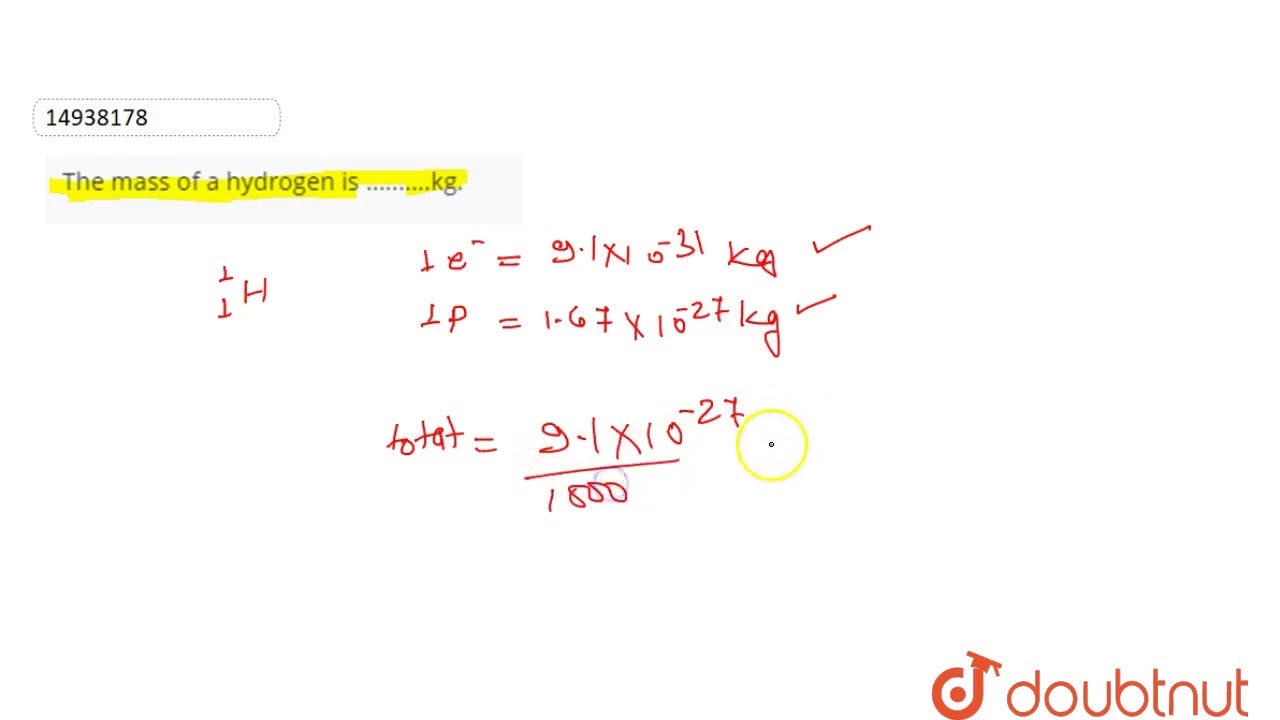
Image taken from the YouTube channel Doubtnut , from the video titled The mass of a hydrogen is ..........kg. .
Hydrogen (H2), the simplest and most abundant element in the universe, is rapidly emerging as a pivotal player in the global energy landscape. Its versatility as both an energy carrier and a vital feedstock for numerous industrial processes positions it as a cornerstone of a sustainable future.
However, unlocking hydrogen's full potential requires a deep understanding of its quantitative requirements. This means accurately estimating the necessary amounts of hydrogen for diverse applications, from powering fuel cells to driving essential chemical reactions.
The Rising Significance of Hydrogen
The increasing importance of hydrogen is intrinsically linked to the global pursuit of a sustainable energy future. As nations strive to decarbonize their economies, hydrogen offers a compelling alternative to fossil fuels across various sectors.
Its ability to be produced from renewable sources like solar and wind power further enhances its appeal. This green hydrogen holds the key to reducing carbon emissions and mitigating climate change.
Why Understanding Hydrogen Quantities Matters
Precisely determining hydrogen quantities is paramount for several reasons. It directly influences the design and optimization of hydrogen-based systems, ensuring efficient resource allocation and cost-effectiveness.
Whether it's calculating the hydrogen needed to power a fleet of fuel cell vehicles or determining the feedstock requirements for ammonia production, accurate estimations are indispensable. These are essential for informed decision-making and strategic planning.
A Guide to Estimating Hydrogen Needs
This article serves as a comprehensive, user-friendly guide to estimating hydrogen needs across a spectrum of applications. It aims to equip readers with the knowledge and tools necessary to navigate the complexities of hydrogen quantification.
By demystifying the factors that influence hydrogen consumption, it empowers engineers, researchers, policymakers, and enthusiasts to make informed decisions and contribute to the advancement of the hydrogen economy.
Who Should Read This?
This guide is tailored for a diverse audience, including:
- Engineers: Designing and implementing hydrogen-based systems.
- Researchers: Investigating hydrogen technologies and applications.
- Policymakers: Developing hydrogen strategies and regulations.
- Enthusiasts: Seeking to deepen their understanding of hydrogen's potential.
Whether you're a seasoned professional or just beginning your journey into the world of hydrogen, this article provides valuable insights and practical guidance for estimating hydrogen needs effectively.
Hydrogen 101: Essential Properties and Principles
Hydrogen (H2), the simplest and most abundant element in the universe, is rapidly emerging as a pivotal player in the global energy landscape. Its versatility as both an energy carrier and a vital feedstock for numerous industrial processes positions it as a cornerstone of a sustainable future.
However, unlocking hydrogen's full potential requires a solid grasp of its fundamental properties. These properties dictate how we produce, store, transport, and utilize this promising energy source. Let's delve into the essential principles that govern hydrogen's behavior.
Understanding Hydrogen's Key Characteristics
Hydrogen, in its diatomic form (H2), exhibits several unique characteristics that differentiate it from other fuels. Understanding these characteristics is paramount in assessing its suitability for various applications.
Molar Mass: The Foundation for Conversions
The molar mass of hydrogen (H2) is approximately 2.016 g/mol or 0.002016 kg/mol. This value represents the mass of one mole of hydrogen molecules.
Molar mass is essential for converting between the mass of hydrogen and the number of moles, a crucial conversion in chemical and engineering calculations.
For instance, if you have 10 grams of hydrogen, you can calculate the number of moles by dividing the mass by the molar mass: 10 g / 2.016 g/mol ≈ 4.96 moles.
Density: A State-Dependent Property
Hydrogen's density varies significantly depending on its state (gas, liquid, or solid), as well as temperature and pressure. In its gaseous form, hydrogen is much less dense than air.
This lower density has important implications for storage and transportation.
The Ideal Gas Law
The density of gaseous hydrogen is heavily influenced by temperature and pressure, as described by the ideal gas law (PV = nRT). Understanding this relationship is crucial for calculating hydrogen density under different conditions.
Standard Conditions
Under standard conditions (STP: 0°C and 1 atm), the density of gaseous hydrogen is approximately 0.08988 g/L. This value serves as a reference point for comparing hydrogen's density under varying conditions.
Specific Energy: The Power Within
Hydrogen boasts a remarkably high specific energy (gravimetric energy density) of approximately 142 MJ/kg. This means that hydrogen stores a significant amount of energy per unit mass, far exceeding that of other common fuels.
For comparison, gasoline has a specific energy of roughly 44-46 MJ/kg. The high specific energy of hydrogen makes it an attractive fuel for applications where weight is a critical factor, such as in transportation.
Volumetric Energy Density: A Storage Challenge
While hydrogen excels in specific energy, its volumetric energy density (energy per unit volume) presents a significant challenge. Due to its low density, hydrogen occupies a large volume in its gaseous state, resulting in a lower volumetric energy density compared to liquid fuels.
This is why hydrogen is often compressed or liquified to reduce its volume for storage and transport. Developing efficient and cost-effective storage solutions that maximize volumetric energy density is a key area of research and development.
Thermodynamics: Understanding Hydrogen Behavior
Thermodynamic principles play a critical role in understanding hydrogen's behavior during production, storage, and utilization. Concepts like enthalpy, entropy, and Gibbs free energy help to predict the feasibility and efficiency of various hydrogen-related processes.
Avogadro's Number: Counting Molecules
Avogadro's number (approximately 6.022 x 10^23) represents the number of molecules in one mole of any substance, including hydrogen.
This fundamental constant is essential for calculating the number of hydrogen molecules involved in chemical reactions and other molecular-level processes.
By understanding these fundamental properties and principles, we gain the necessary foundation to navigate the complexities of hydrogen production, storage, and utilization, paving the way for its successful integration into a sustainable energy future.
From Production to Kilograms: A Look at Hydrogen Generation
Hydrogen (H2), the simplest and most abundant element in the universe, is rapidly emerging as a pivotal player in the global energy landscape. Its versatility as both an energy carrier and a vital feedstock for numerous industrial processes positions it as a cornerstone of a sustainable future.
However, unlocking its full potential hinges on efficient and scalable production methods. This section delves into the major pathways for hydrogen generation, examining how each process influences the final yield in kilograms and emphasizing critical efficiency considerations.
Electrolysis: Splitting Water with Electricity
Electrolysis represents a promising route to green hydrogen, leveraging electricity to split water (H2O) into its constituent elements: hydrogen and oxygen. This method holds significant appeal due to its potential for zero-emission operation when powered by renewable energy sources.
Several types of electrolysis technologies exist, each with its own characteristics and performance profiles:
-
Alkaline Electrolysis: A mature technology using an alkaline electrolyte (e.g., potassium hydroxide). It is generally cost-effective but can have lower current densities compared to other methods.
-
Proton Exchange Membrane (PEM) Electrolysis: Employs a solid polymer electrolyte, enabling higher current densities and more dynamic operation. PEM electrolyzers are well-suited for integration with intermittent renewable energy sources.
-
Solid Oxide Electrolysis Cell (SOEC): Operates at high temperatures, potentially increasing efficiency and allowing for the use of waste heat. However, SOEC technology is still under development and faces challenges related to material durability.
Estimating hydrogen production from electrolysis requires careful consideration of efficiency factors. A typical electrolyzer consumes electricity to drive the water-splitting reaction. The efficiency of the electrolyzer determines how much hydrogen is produced per unit of electricity input (kWh).
For instance, a modern PEM electrolyzer might achieve an efficiency of 60-80%. This means that for every kWh of electricity consumed, a certain amount of hydrogen is generated. The exact quantity can be calculated based on the electrolyzer's specific characteristics and operating conditions.
Steam Methane Reforming (SMR): A Mature Technology
Steam methane reforming (SMR) is the most widely used method for hydrogen production today. This process involves reacting methane (CH4), the primary component of natural gas, with steam (H2O) at high temperatures and pressures in the presence of a catalyst.
The main inputs for SMR are methane and steam, while the primary outputs are hydrogen (H2) and carbon dioxide (CO2). The chemical reaction can be represented as:
CH4 + H2O → CO + 3H2
CO + H2O → CO2 + H2
Although SMR is a cost-effective method, it results in significant carbon dioxide emissions. Carbon capture and storage (CCS) technologies can be integrated to mitigate these emissions, but this adds complexity and cost to the process.
Estimating hydrogen production from SMR involves calculating the mass of hydrogen produced based on the amount of methane input. The efficiency of the SMR process is a crucial factor, reflecting the ratio of hydrogen energy output to methane energy input.
Typical SMR plants achieve efficiencies of 70-90%. This means that a certain mass of methane will yield a corresponding mass of hydrogen, taking into account the efficiency of the reforming process and the molar masses of the reactants and products.
Other Production Methods: A Glimpse into the Future
While electrolysis and SMR dominate the hydrogen production landscape, several other emerging technologies hold promise for the future. These methods offer alternative pathways to hydrogen generation, each with unique advantages and challenges.
-
Biomass Gasification: This process converts biomass materials (e.g., wood, agricultural residues) into a syngas, which can then be processed to produce hydrogen.
-
Photoelectrochemical Water Splitting: This method uses sunlight and semiconductor materials to directly split water into hydrogen and oxygen.
-
Methane Pyrolysis: Decomposes methane into hydrogen and solid carbon.
While these alternative methods are still in various stages of development, they represent important avenues for diversifying hydrogen production and potentially reducing environmental impacts. However, their scalability, efficiency, and cost-effectiveness remain key areas of focus for ongoing research and development efforts.
Taming the Molecule: Hydrogen Storage Solutions
Having explored the nuances of hydrogen generation, the next crucial step lies in effectively storing this versatile energy carrier. The method of storage significantly impacts the overall efficiency and practicality of hydrogen as an energy solution. This section delves into the various technologies employed to store hydrogen, examining how each affects volumetric and gravimetric densities, ultimately influencing the amount of usable hydrogen available for downstream applications.
The Critical Role of Hydrogen Storage Tanks
The unsung heroes of hydrogen storage are the tanks themselves. The materials used in their construction, along with their design, play a pivotal role in determining both volumetric and gravimetric densities, as well as the overall safety and longevity of the storage system.
Material Selection:
Advanced composite materials, such as carbon fiber reinforced polymers, are increasingly favored for their high strength-to-weight ratio. These materials allow for lighter tanks, improving gravimetric density, which is especially critical in transportation applications.
Tank Design:
Tank design, particularly the shape and layering of materials, is crucial for withstanding the extreme pressures involved in compressed hydrogen storage. Cylindrical and spherical designs are common, each offering different advantages in terms of stress distribution and space utilization.
Compressed Hydrogen: High Pressure, High Potential
One of the most established methods for storing hydrogen involves compressing it to high pressures. This approach increases the volumetric density of hydrogen, allowing more of it to be stored in a given volume.
Advantages of Compressed Hydrogen:
- Relatively mature technology.
- Faster refueling times compared to other methods.
Disadvantages of Compressed Hydrogen:
- Lower volumetric energy density compared to liquid hydrogen.
- High energy consumption for compression.
- Potential safety concerns associated with high-pressure systems.
Pressure Ranges:
Typical pressure ranges for compressed hydrogen storage are 350 bar (5,000 psi) and 700 bar (10,000 psi). Higher pressures enable greater storage capacity but also require more robust and expensive tank designs.
Liquid Hydrogen: The Cryogenic Frontier
Another prominent storage method involves liquefying hydrogen at extremely low temperatures (-253°C or -423°F). This cryogenic storage method significantly increases the volumetric energy density compared to compressed hydrogen.
Cryogenic Storage:
Liquefaction drastically reduces the volume required for storage. This makes liquid hydrogen suitable for applications where space is a constraint, such as in aircraft or large-scale energy storage facilities.
Energy Intensive Liquefaction:
The liquefaction process is energy-intensive, consuming a significant portion of the energy content of the hydrogen itself. This energy penalty needs to be carefully considered when evaluating the overall efficiency of liquid hydrogen storage.
Boil-Off Losses:
Even with highly insulated tanks, liquid hydrogen experiences boil-off losses due to heat ingress. These losses, where hydrogen evaporates and vents, reduce the amount of usable hydrogen over time.
Other Storage Methods: A Glimpse into the Future
While compressed and liquid hydrogen storage are the most prevalent, research and development efforts are focused on alternative methods that could offer improved performance and safety characteristics.
Metal Hydrides:
These materials absorb hydrogen into their crystalline structure, offering a safer and more compact storage solution compared to compressed hydrogen. However, metal hydrides often suffer from low gravimetric density and slow absorption/desorption rates.
Chemical Hydrogen Storage:
This approach involves chemically bonding hydrogen to other molecules, such as ammonia or liquid organic hydrogen carriers (LOHCs). Chemical storage offers high volumetric density and stability, but requires energy-intensive release processes.
The selection of the appropriate hydrogen storage method hinges on a multitude of factors, including application requirements, cost considerations, and safety protocols. As technology advances and infrastructure develops, expect to see continued innovation in hydrogen storage, further unlocking the potential of this clean energy carrier.
Hydrogen in Action: Applications Across Industries
Having secured viable methods of hydrogen storage, a crucial question arises: How is this stored hydrogen actually used? The breadth of hydrogen applications spans diverse sectors, each with unique requirements and consumption patterns.
Understanding these applications is paramount to accurately estimating hydrogen demand and deploying resources effectively. This section will explore several key areas where hydrogen is making a significant impact.
Fuel Cells: Powering the Future with Hydrogen
Fuel cells represent one of the most promising applications of hydrogen, offering a clean and efficient pathway to electricity generation.
These electrochemical devices convert the chemical energy of hydrogen directly into electricity, with water and heat as the primary byproducts. This process is significantly cleaner than traditional combustion-based power generation.
Types of Fuel Cells
Several types of fuel cells exist, each with distinct characteristics and suitability for specific applications. Proton Exchange Membrane Fuel Cells (PEMFCs) are commonly used in transportation and portable power applications due to their low operating temperature and high power density.
Solid Oxide Fuel Cells (SOFCs), on the other hand, operate at higher temperatures and are well-suited for stationary power generation and combined heat and power (CHP) systems.
Hydrogen Consumption in Fuel Cells
The amount of hydrogen consumed by a fuel cell depends on its efficiency and power output. Generally, fuel cells require approximately 1 kg of hydrogen to generate 33.3 kWh of electricity. This translates to roughly 0.03 kg of hydrogen per kWh. These figures are very much dependent on cell type and operating conditions.
Hydrogen Vehicles (FCVs): A Zero-Emission Transportation Solution
Hydrogen fuel cell vehicles (FCVs) offer a compelling alternative to traditional internal combustion engine vehicles and battery electric vehicles (BEVs).
FCVs utilize fuel cells to convert hydrogen into electricity, which then powers an electric motor. The only emission from an FCV is water vapor, making them a true zero-emission transportation solution.
Hydrogen Tank Capacity and Range
FCVs typically have hydrogen tanks with a capacity of around 5-8 kg, providing a driving range of 300-400 miles.
The range depends heavily on driving style, road conditions, and vehicle efficiency.
Hydrogen Consumption Rates
Hydrogen consumption rates in FCVs vary depending on driving conditions. In general, FCVs consume approximately 1 kg of hydrogen per 60-100 miles. Aggressive driving or driving in stop-and-go traffic will increase consumption, while highway driving at a constant speed will improve fuel economy.
Industrial Feedstock: A Cornerstone of Chemical Processes
Hydrogen plays a crucial role as an industrial feedstock in various chemical processes, including ammonia production and oil refining.
These industries consume large quantities of hydrogen, making them significant drivers of hydrogen demand.
Ammonia Production
Ammonia (NH3) production is one of the largest consumers of hydrogen globally. Hydrogen is combined with nitrogen through the Haber-Bosch process to produce ammonia, a key ingredient in fertilizers. The production of one ton of ammonia requires approximately 176 kg of hydrogen.
Oil Refining
In oil refineries, hydrogen is used in hydrocracking and hydrotreating processes to remove impurities and upgrade the quality of crude oil.
The amount of hydrogen needed for refining varies depending on the crude oil composition and the desired product specifications.
Energy Storage: Harnessing Hydrogen for Grid Stability
Hydrogen offers a promising solution for large-scale energy storage, particularly for integrating intermittent renewable energy sources such as solar and wind power.
The "power-to-gas" concept involves using excess renewable energy to produce hydrogen via electrolysis. The hydrogen can then be stored and used later to generate electricity or injected into natural gas pipelines.
Round-Trip Efficiency
One of the key considerations for hydrogen energy storage is the round-trip efficiency, which represents the percentage of energy that can be recovered after storage and conversion. Current power-to-gas systems have a round-trip efficiency of around 30-50%. Improvements in electrolysis and fuel cell technologies are needed to improve efficiency.
Power Generation: Hydrogen-Fueled Turbines
Hydrogen can also be used directly in power plants as a fuel for turbines.
Hydrogen-fueled turbines offer a clean and flexible way to generate electricity, especially during peak demand or when renewable energy sources are unavailable.
While still in early stages of development, hydrogen-fueled turbines have the potential to play a significant role in decarbonizing the power sector.
Ultimately, understanding the specific hydrogen needs across these diverse applications is crucial for planning and deploying hydrogen infrastructure effectively. As technology advances and the demand for clean energy grows, hydrogen is poised to play an increasingly important role in shaping a sustainable future.
The Big Picture: Factors Influencing Your Hydrogen Needs
Having explored the diverse applications of hydrogen, it becomes clear that a multitude of factors influence its consumption and demand. Accurately estimating hydrogen needs requires a holistic approach, taking into account not only the desired application but also the nuances of its implementation. Efficiency, storage, and purity all play critical roles in determining the true hydrogen demand.
Application Efficiency: Maximizing Hydrogen's Potential
The efficiency of the chosen application directly impacts the amount of hydrogen required to achieve a specific outcome. Whether it's generating electricity via fuel cells or producing ammonia in an industrial plant, understanding the conversion rate is paramount.
Fuel cell efficiency, for instance, dictates how much hydrogen is needed to produce a kilowatt-hour of electricity. Higher efficiency translates to lower hydrogen consumption and reduced operational costs.
Similarly, the yield of an industrial process determines the amount of hydrogen required to produce a unit of output. Optimizing process parameters and employing advanced technologies can significantly improve yield and minimize hydrogen demand.
Storage Method: Preserving Hydrogen's Integrity
The method used to store hydrogen significantly impacts the amount of usable hydrogen available for application. Different storage technologies exhibit varying volumetric and gravimetric densities, affecting the overall amount of hydrogen that can be stored in a given space or weight.
Furthermore, storage methods can introduce losses, such as boil-off in liquid hydrogen storage or energy consumption for compression. These losses must be factored into the estimation process to avoid underestimating hydrogen needs.
Choosing the right storage method is therefore critical for maximizing the efficiency of the hydrogen supply chain.
Purity Requirements: Meeting Application Demands
The required purity of hydrogen varies depending on the application. Fuel cells, for example, often require high-purity hydrogen to prevent degradation of the fuel cell stack.
Impurities such as carbon monoxide and sulfur can poison the catalyst and reduce fuel cell performance.
Industrial processes may have different purity requirements, depending on the specific reaction chemistry. Failing to meet the required purity can lead to process inefficiencies, equipment damage, or even safety hazards. Therefore, accurately assessing purity requirements is essential for selecting the appropriate hydrogen source and purification technologies.
Environmental Factors: Temperature and Pressure
Environmental conditions such as temperature and pressure also influence the behavior of hydrogen. These factors affect hydrogen density and storage capacity.
For example, changes in temperature can lead to pressure fluctuations in compressed hydrogen storage tanks. Extreme temperatures can also impact the performance of fuel cells and other hydrogen-based technologies.
Therefore, it's important to consider the operating environment when estimating hydrogen needs. By carefully evaluating these factors, engineers, researchers, and policymakers can ensure that hydrogen systems are designed and operated efficiently, safely, and reliably.
The Bottom Line: Economic Considerations for Hydrogen
Having explored the diverse applications of hydrogen, it becomes clear that a multitude of factors influence its consumption and demand. Accurately estimating hydrogen needs requires a holistic approach, taking into account not only the desired application but also the nuances of its implementation.
However, technical feasibility is only one piece of the puzzle. The economic viability of hydrogen projects hinges critically on understanding the interplay between production costs and market dynamics. This section delves into these economic considerations, highlighting their profound impact on the widespread adoption of hydrogen technologies.
The Decisive Role of Hydrogen Production Costs
The cost of producing hydrogen is a pivotal factor shaping its adoption across various sectors. High production costs can significantly hinder the economic feasibility of hydrogen projects, even if the underlying technology is sound.
Ultimately, production cost exerts a direct influence on crucial decision-making. Stakeholders, whether they are investors, project developers, or end-users, carefully weigh the cost of hydrogen against competing alternatives.
A cost-prohibitive hydrogen supply will inevitably lead to the selection of more economically attractive energy solutions, irrespective of the environmental or performance benefits that hydrogen may offer. This makes cost reduction in hydrogen production paramount to achieving widespread market penetration.
Comparing Production Methods
The cost of producing hydrogen varies significantly depending on the production method employed. Steam Methane Reforming (SMR), currently the most prevalent method, typically boasts lower production costs compared to other technologies like electrolysis.
However, SMR's reliance on fossil fuels introduces carbon emissions, prompting a shift towards cleaner production pathways. Electrolysis, using renewable energy sources, offers a pathway to "green" hydrogen, but it often comes with a higher price tag.
The economic trade-offs between different production methods are constantly evolving as technology advances and renewable energy costs decline.
Furthermore, it also depends on the energy resource prices that feed into these technologies, whether it is natural gas, or renewable electricity. These price fluctuations also influence the economic competitiveness of hydrogen.
Therefore, understanding these nuances is crucial for stakeholders evaluating the economic viability of hydrogen projects and making informed decisions regarding technology selection and investment strategies.
Navigating Hydrogen Market Prices
Beyond production costs, hydrogen market prices play a crucial role in determining the economic viability of hydrogen projects. These prices, reflecting supply and demand dynamics, directly influence the financial returns of hydrogen production and utilization.
Therefore, these market prices are a major decision point for investing in hydrogen technologies.
Price Disparities and Regional Variations
Hydrogen market prices are not uniform; they vary significantly across different regions due to several factors, including:
- Local energy prices
- Infrastructure availability
- Governmental support
- Regional demand.
Understanding these regional price variations is essential for project developers seeking to optimize their investment decisions and identify the most favorable markets for hydrogen production and deployment.
Accessing reliable and up-to-date market price information is critical for accurate financial modeling and risk assessment in the hydrogen sector.
Price Discovery and Market Maturity
The hydrogen market is still relatively nascent compared to mature energy markets like oil and natural gas. Consequently, price discovery mechanisms are still developing, and price transparency can be limited in certain regions.
As the hydrogen market matures, it's anticipated that more robust price indices and trading platforms will emerge, providing greater transparency and facilitating more efficient price discovery. This increased transparency will be crucial for fostering investor confidence and driving market growth.
Navigating the Rules: Regulatory Landscape and Standards
Having explored the economic considerations of hydrogen, it is crucial to acknowledge the regulatory landscape that governs its production, storage, and utilization. Adhering to established guidelines and standards is not merely a matter of compliance but a fundamental aspect of ensuring safe, responsible, and sustainable hydrogen deployment. This section will explore the vital roles of standards organizations and the application of relevant standards across various sectors.
The Role of Standards Organizations
International standards organizations play a pivotal role in shaping the hydrogen economy. These organizations provide frameworks for ensuring safety, quality, and interoperability across the hydrogen value chain. Their work is crucial for fostering trust and confidence among stakeholders, paving the way for wider adoption.
Some of the most prominent organizations include:
-
ISO (International Organization for Standardization): ISO develops international standards covering a wide range of aspects related to hydrogen, including production, storage, transportation, and use.
-
SAE International (formerly Society of Automotive Engineers): SAE focuses on developing standards for hydrogen-powered vehicles, fueling systems, and related components.
-
ASTM International (formerly American Society for Testing and Materials): ASTM develops consensus standards for materials, products, systems, and services, including those related to hydrogen.
These organizations work collaboratively with industry experts, government agencies, and research institutions to develop comprehensive standards that address key challenges and promote best practices. The consistent application of these standards is essential for ensuring safety and facilitating international trade.
Standards for Hydrogen Fueling Stations
The deployment of hydrogen fueling stations requires strict adherence to safety standards to protect both operators and the public. Standards cover various aspects, including:
-
Equipment Safety: Standards specify requirements for hydrogen dispensers, compressors, storage tanks, and other critical equipment to ensure they are designed and operated safely.
-
Operating Procedures: Standards outline safe operating procedures for fueling vehicles, handling hydrogen, and responding to emergencies.
-
Safety Distances: Standards define minimum safety distances between fueling station components and surrounding buildings or infrastructure.
-
Emergency Shutdown Systems: Standards require the installation of emergency shutdown systems to quickly isolate the hydrogen supply in case of an accident.
-
Personnel Training: Standards specify training requirements for fueling station operators to ensure they are competent in handling hydrogen and responding to emergencies.
Compliance with these standards is essential for minimizing risks and ensuring the safe operation of hydrogen fueling stations. Regular inspections and audits are necessary to verify compliance and identify potential safety hazards.
Vehicle Safety Standards
Hydrogen-powered vehicles must meet stringent safety standards to protect occupants and the public. These standards cover various aspects, including:
-
Hydrogen Tank Integrity: Standards specify requirements for the design, construction, and testing of hydrogen storage tanks to ensure they can withstand extreme pressures and temperatures.
-
Fuel Cell System Safety: Standards address the safety of fuel cell systems, including measures to prevent hydrogen leaks, electrical hazards, and thermal runaway.
-
Vehicle Crash Testing: Hydrogen-powered vehicles must undergo rigorous crash testing to ensure they provide adequate protection to occupants in the event of a collision.
-
Hydrogen Leak Detection: Standards require the installation of hydrogen leak detection systems to alert occupants and emergency responders in case of a leak.
-
Ventilation Systems: Standards specify requirements for ventilation systems to prevent the accumulation of hydrogen in enclosed spaces.
-
Material Compatibility: Ensure there is proper selection of materials compatible with hydrogen to prevent embrittlement or degradation.
These standards are constantly evolving to incorporate new technologies and address emerging safety concerns. Automakers must demonstrate compliance with these standards before they can sell hydrogen-powered vehicles.
Industrial Applications: Setting the Bar for Safety
The use of hydrogen in industrial processes also requires strict adherence to safety standards to protect workers and the environment. These standards cover various aspects, including:
-
Equipment Design and Operation: Standards specify requirements for the design, construction, and operation of hydrogen-using equipment, such as reactors, compressors, and storage tanks.
-
Ventilation and Leak Detection: Standards require the installation of adequate ventilation systems and hydrogen leak detection systems to prevent the accumulation of hydrogen in enclosed spaces.
-
Flammability and Explosion Protection: Standards outline measures to prevent hydrogen fires and explosions, such as using intrinsically safe equipment and implementing proper grounding procedures.
-
Emergency Response Planning: Standards require the development of comprehensive emergency response plans to address potential hydrogen-related incidents.
-
Process Safety Management (PSM): Implementation of PSM principles to identify, evaluate, and control process hazards involving hydrogen.
-
Material Selection: Choosing appropriate materials compatible with hydrogen to avoid embrittlement, corrosion, or other degradation issues.
Compliance with these standards is essential for minimizing risks and ensuring the safe use of hydrogen in industrial settings. Regular audits and inspections are necessary to verify compliance and identify potential safety hazards.
By adhering to these regulatory frameworks and safety standards, the hydrogen economy can progress responsibly, mitigating risks and fostering public confidence in its long-term viability. As the industry matures, continuous refinement and adaptation of these guidelines will be essential to ensure safety, efficiency, and environmental sustainability.
Innovation and Research: Shaping the Future of Hydrogen
Having navigated the regulatory landscape and standards governing hydrogen, it's vital to acknowledge the pivotal role of research and development (R&D) organizations in propelling advancements in hydrogen technologies and infrastructure. These institutions are instrumental in driving innovation and accelerating the widespread adoption of hydrogen as a sustainable energy carrier.
The U.S. Department of Energy (DOE) and National Renewable Energy Laboratory (NREL)
The U.S. Department of Energy (DOE) and the National Renewable Energy Laboratory (NREL) stand as cornerstones of hydrogen research and development.
The DOE's Hydrogen and Fuel Cell Technologies Office, for example, strategically invests in projects aimed at reducing the cost and improving the performance of hydrogen production, storage, and utilization technologies.
NREL, as a leading national laboratory, conducts cutting-edge research in areas such as electrolysis, hydrogen storage materials, and fuel cell development. Their contributions are essential for de-risking and validating novel hydrogen technologies.
NREL's work spans the entire spectrum of hydrogen from basic materials science to system-level demonstrations. They are an essential resource for industry and government stakeholders alike.
Global Research Institutions and Consortia
Beyond the DOE and NREL, a global network of research institutions and industry consortia is actively shaping the future of hydrogen.
Universities around the world conduct fundamental research on novel materials, advanced catalysts, and innovative production pathways.
Examples include but are not limited to:
- The University of Tokyo (Japan).
- Delft University of Technology (Netherlands).
- Helmholtz-Zentrum Hereon (Germany).
- Many more.
Industry consortia, such as the Hydrogen Council, bring together leading companies to accelerate the deployment of hydrogen technologies through collaboration and knowledge sharing. These partnerships are critical for bridging the gap between laboratory research and commercial applications.
These consortia also play a vital role in advocating for supportive policies and regulations that can foster the growth of the hydrogen economy.
Key Areas of Ongoing Research
Ongoing research efforts are focused on addressing key challenges and unlocking new opportunities for hydrogen.
- Advanced Electrolysis Technologies: Research is focused on next-generation electrolyzers capable of achieving higher efficiencies and lower costs.
- Novel Storage Materials: Scientists are exploring new materials for hydrogen storage, aiming for higher energy densities and improved safety.
- Hydrogen Production from Renewable Resources: Developing sustainable pathways for hydrogen production using solar, wind, and biomass.
- Fuel Cell Durability and Performance: Improving the lifespan and performance of fuel cells for transportation, stationary power, and other applications.
These efforts are crucial for making hydrogen a more competitive and widely adopted energy solution.
The Path Forward
The future of hydrogen hinges on sustained investment in research and development. Continued innovation is essential for driving down costs, improving performance, and unlocking the full potential of hydrogen as a clean and versatile energy carrier. By fostering collaboration between research institutions, industry, and government, we can accelerate the transition to a sustainable hydrogen economy.
Video: Kg of Hydrogen: How Much Do You Need?
FAQs: Kg of Hydrogen: How Much Do You Need?
What determines how many kg of hydrogen I need for a fuel cell vehicle?
The mass of hydrogen in kg required depends on the vehicle's range and fuel efficiency. Longer range and lower efficiency will require a greater mass of hydrogen in kg to be stored on board.
How does the mass of hydrogen in kg compare to gasoline for the same energy?
Hydrogen has a much lower energy density by volume than gasoline. You need significantly more volume of hydrogen to achieve the same energy output as a smaller volume of gasoline. This translates to a greater mass of hydrogen in kg required to achieve the same range.
How does the pressure at which hydrogen is stored affect how much mass of hydrogen in kg I can fit in a tank?
Higher storage pressures allow for greater density of hydrogen. This means you can fit more mass of hydrogen in kg into a tank of a given volume compared to lower pressure storage, ultimately increasing range.
Besides fuel cell vehicles, what other applications need a specific mass of hydrogen in kg?
Beyond vehicles, many industries use hydrogen. These include industrial processes (like ammonia production), energy storage, and potentially even heating. The required mass of hydrogen in kg will depend on the scale and efficiency of the specific application.
So, there you have it! Whether you're fueling a car, powering a factory, or just plain curious, understanding how much mass of hydrogen in kg you actually need is key. Hopefully, this gave you a clearer picture of the numbers involved and helps you on your hydrogen journey.