Freezing Point of Hydrogen: Uses & Safety Guide
Hydrogen, a crucial element in the development of technologies supported by organizations such as NASA, exhibits a freezing point of hydrogen at approximately -259.14 degrees Celsius, a critical parameter for its storage and handling. Cryogenic storage tanks, specialized containers engineered to maintain extremely low temperatures, are essential for preserving hydrogen in its liquid state, a necessity given this freezing point. Proper handling procedures and adherence to safety guidelines are paramount when working with liquid hydrogen to mitigate risks associated with its flammability, which the National Fire Protection Association (NFPA) addresses with detailed standards. Understanding the freezing point of hydrogen is fundamental for applications ranging from rocket propulsion to advanced materials research, highlighting its role in innovative fields explored by scientists like James Dewar, a pioneer in cryogenic research.
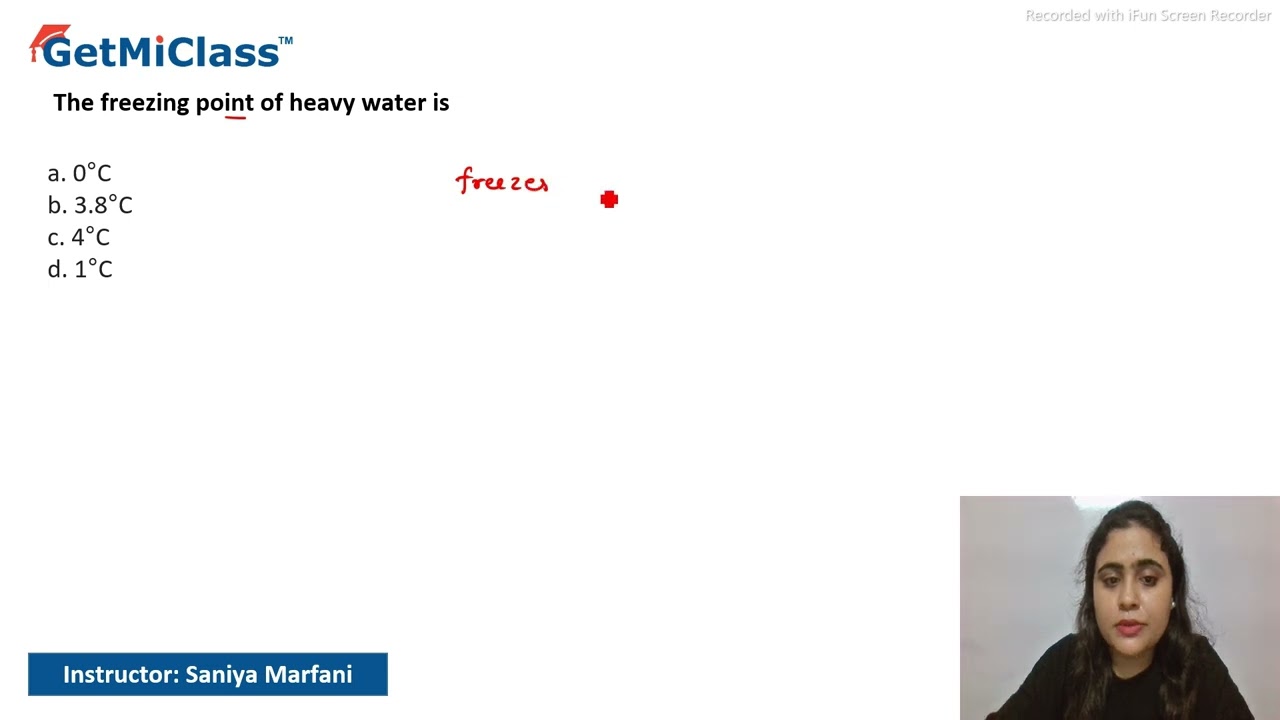
Image taken from the YouTube channel GetMiClass , from the video titled Freezing point KCET 11th Chemistry Hydrogen .
Unveiling the Freezing Point of Hydrogen: A Fundamental Property
The freezing point of hydrogen, a seemingly simple concept, unlocks a gateway to understanding the behavior of matter at extreme cryogenic conditions. It serves as a cornerstone in the realm of thermophysical properties, dictating its interactions and suitability across a spectrum of scientific and industrial applications.
Defining the Freezing Point of Hydrogen
The freezing point, also known as the solidification point, is the temperature at which a liquid transforms into a solid state at a given pressure.
For hydrogen, this transition occurs at approximately 14.01 Kelvin (-259.14 degrees Celsius or -434.45 degrees Fahrenheit) at standard atmospheric pressure. This temperature represents the equilibrium point where the liquid and solid phases of hydrogen can coexist. Precise measurement and understanding are crucial for various applications.
Significance in Thermophysical Studies and Applications
The freezing point of hydrogen holds immense significance across diverse fields:
-
Thermophysical Studies: It provides a critical data point for developing and validating equations of state and thermodynamic models. These models are essential for predicting hydrogen's behavior under varying conditions.
-
Cryogenic Engineering: Understanding this property is paramount in designing and operating cryogenic systems. These systems are for liquefaction, storage, and transportation of hydrogen.
-
Aerospace Applications: Liquid hydrogen, due to its low freezing point and high energy density, is a preferred rocket propellant. Precise temperature control is vital for efficient and safe operation.
-
Fundamental Research: The study of hydrogen's phase transitions at ultra-low temperatures allows insights into quantum mechanics. Specifically relating to molecular interactions and condensed matter physics.
Scope of Discussion: Liquid-to-Solid Transition and Influential Factors
This discussion focuses primarily on the liquid-to-solid phase transition of hydrogen. We will explore the following key areas:
-
Phase Transition Mechanism: Investigating the process by which liquid hydrogen transforms into its solid form. Focusing on the energy dynamics involved.
-
Influential Factors: Examining parameters such as pressure and isotopic composition, that can alter the freezing point. These parameters play a critical role in how it behaves.
-
Cryogenic Handling: Reviewing essential considerations for safely handling hydrogen at cryogenic temperatures. This is paramount to avoid any accidental hazards.
By understanding these aspects, we can fully appreciate the critical role of the freezing point of hydrogen. We can also explore its applications in pushing the boundaries of science and technology.
Fundamentals: Phase Transition and Unique Properties of Hydrogen
Understanding the freezing point of hydrogen necessitates a firm grasp of the fundamental principles governing phase transitions and the peculiar behavior of hydrogen at cryogenic temperatures. This section will explore the liquid-to-solid phase transition, differentiate between melting and freezing points, and discuss the distinctive properties of hydrogen, particularly in its solid form, which is critical for a comprehensive understanding.
Phase Transition: Liquid to Solid
The transition from a liquid to a solid state, commonly known as freezing, is a phase transition where a substance changes from a liquid to a solid upon cooling. This occurs when the temperature of the liquid decreases to the point where the kinetic energy of the molecules is no longer sufficient to overcome the intermolecular forces holding them apart.
As the temperature drops, the molecules slow down and pack more closely together. At the freezing point, these intermolecular attractions become dominant, causing the molecules to arrange themselves into a fixed, ordered structure characteristic of a solid.
Distinguishing Melting Point and Freezing Point
While often used interchangeably, the melting point and freezing point represent the same temperature for a given substance under specific conditions, but refer to opposing processes. The melting point is the temperature at which a solid transforms into a liquid, while the freezing point is the temperature at which a liquid transforms into a solid.
For pure crystalline substances, these points are identical. However, in some amorphous materials or mixtures, a freezing range may be observed rather than a sharp freezing point. The freezing point can also be affected by factors such as supercooling, where a liquid is cooled below its freezing point without solidifying.
Properties of Solid Hydrogen: Ortho- and Para-Hydrogen
Solid hydrogen exhibits unique properties due to the existence of two distinct forms: ortho-hydrogen and para-hydrogen. These forms arise from the different spin orientations of the two protons in the hydrogen molecule.
Ortho-hydrogen has parallel proton spins (total spin = 1), while para-hydrogen has anti-parallel proton spins (total spin = 0). At room temperature, hydrogen exists as a mixture of approximately 75% ortho-hydrogen and 25% para-hydrogen.
However, as hydrogen is cooled towards its freezing point, the equilibrium shifts towards the para-hydrogen form, which is the lower energy state. The conversion from ortho- to para-hydrogen is an exothermic process, releasing heat.
This heat release can cause significant problems during the storage and handling of liquid hydrogen, as it can lead to boil-off losses. Therefore, a catalyst, such as iron oxide, is typically used to accelerate the conversion to para-hydrogen before liquefaction or solidification.
Density, Thermal Conductivity, and Heat Capacity at Cryogenic Temperatures
At cryogenic temperatures, the density of solid hydrogen is significantly higher than that of gaseous hydrogen. This increased density makes solid hydrogen an attractive medium for high-density storage.
The thermal conductivity of solid hydrogen is also temperature-dependent. Initially, as the temperature decreases, the thermal conductivity increases due to reduced phonon scattering. However, at very low temperatures, the thermal conductivity decreases again due to boundary scattering and other quantum effects.
The heat capacity of solid hydrogen also exhibits unique behavior at cryogenic temperatures. At very low temperatures, the heat capacity approaches zero, as predicted by the third law of thermodynamics. The heat capacity is also affected by the ortho-para composition, with para-hydrogen having a lower heat capacity than ortho-hydrogen.
The Role of Intermolecular Forces
The phase transition from liquid to solid hydrogen is governed by weak intermolecular forces, primarily van der Waals forces, specifically London dispersion forces. These forces arise from temporary fluctuations in electron distribution, creating transient dipoles that induce dipoles in neighboring molecules.
These forces are relatively weak compared to chemical bonds, but they become significant at low temperatures when the kinetic energy of the molecules is reduced. The strength of these van der Waals forces determines the freezing point of hydrogen, which is exceptionally low compared to other substances.
At temperatures near the freezing point, these intermolecular forces are strong enough to overcome the kinetic energy of the hydrogen molecules, causing them to lock into a crystalline lattice structure, forming solid hydrogen. Understanding these interactions is critical to predicting and controlling the behavior of hydrogen in cryogenic applications.
Influencing Factors: Pressure and Isotopic Effects on Freezing Point
Having established a foundation in the fundamental properties of hydrogen, we now turn our attention to the external influences that can significantly alter its freezing point. Specifically, we will examine the roles of pressure and isotopic composition, two key factors that deviate the freezing point from its standard value.
The Influence of Pressure on Hydrogen's Freezing Point
The freezing point of any substance, including hydrogen, is intrinsically linked to pressure. As pressure increases, the freezing point generally increases as well. This phenomenon arises from the fact that higher pressure favors the denser phase of a substance, which is typically the solid phase.
In the case of hydrogen, the application of external pressure compresses the liquid, driving the molecules closer together. This closer proximity strengthens the intermolecular forces (van der Waals forces), making it more energetically favorable for the liquid to transition into the solid state.
Pressure-Temperature Phase Diagrams
To illustrate the relationship between pressure and the freezing point of hydrogen, we rely on pressure-temperature (P-T) phase diagrams. These diagrams provide a visual representation of the different phases of hydrogen (solid, liquid, gas) and the conditions under which they are stable.
The solid-liquid coexistence curve, also known as the melting curve, depicts the freezing point of hydrogen as a function of pressure. By examining this curve, one can readily determine the freezing point at any given pressure. The slope of the curve dictates how sensitive the freezing point is to changes in pressure.
Isotopic Effects: The Role of Deuterium and Tritium
Hydrogen exists in nature as a mixture of isotopes, primarily protium (¹H), deuterium (²H or D), and tritium (³H or T). While these isotopes share the same electronic structure, their differing masses give rise to subtle but significant differences in their physical properties, including their freezing points.
Freezing Points of Hydrogen Isotopes
Deuterium and tritium, being heavier than protium, exhibit higher freezing points. This is largely due to the effects of mass on the vibrational energy of the molecules. Heavier isotopes have lower vibrational frequencies, which translates to a reduced zero-point energy.
The reduced zero-point energy makes the solid phase more stable relative to the liquid phase for heavier isotopes. Consequently, a higher temperature is required to overcome the intermolecular forces and induce melting, thus resulting in a higher freezing point.
Mass Variation and Freezing Point Differences
The differences in freezing points among hydrogen isotopes are directly correlated with their mass variations. The greater the mass difference, the more pronounced the difference in freezing points. Therefore, tritium, being the heaviest isotope, has the highest freezing point, followed by deuterium, and then protium.
These isotopic effects are important considerations in various applications, particularly in cryogenic engineering and nuclear fusion research, where the precise control and understanding of hydrogen's phase behavior are paramount. Understanding the influence of isotopic composition is vital when dealing with mixtures of hydrogen isotopes.
Cryogenics: Unlocking Hydrogen's Potential at Ultra-Low Temperatures
Having examined the factors influencing the freezing point of hydrogen, it is now crucial to understand the broader scientific domain that enables its study and utilization: cryogenics. This field is not merely a passive observer; it is an active enabler, providing the tools and techniques necessary to probe the behavior of hydrogen at its most extreme thermal states.
Cryogenics, fundamentally, is the science of very low temperatures. It encompasses a range of disciplines, from physics and chemistry to materials science and engineering, all focused on understanding and manipulating matter at temperatures far below those encountered in everyday life.
Its relevance to the study of hydrogen's freezing point is paramount. Without cryogenic techniques, reaching and maintaining the temperatures required to solidify hydrogen would be impossible.
The Indispensable Role of Cryogenics
The study of cryogenics provides the means to carefully examine hydrogen's unusual properties. It enables the observation of the delicate phase transitions, and helps to measure its behavior as it reaches its freezing point, and transitions into its solid form.
Liquid Hydrogen as Rocket Propellant
One of the most well-established applications of cryogenic hydrogen is its use as a high-energy rocket propellant. In this application, liquid hydrogen is combined with liquid oxygen to produce a powerful and efficient combustion reaction, capable of generating the immense thrust needed to propel rockets into space.
Its low molecular weight and high energy content per unit mass make it an ideal fuel for space exploration.
The use of liquid hydrogen as a rocket propellant relies heavily on cryogenic engineering to safely store, transfer, and manage the fuel at extremely low temperatures.
Solid Hydrogen: A Future Storage Medium?
While liquid hydrogen is already widely used, solid hydrogen holds significant potential as a high-density storage medium. Solid hydrogen offers the prospect of storing more hydrogen in a given volume compared to liquid or gaseous forms. This compact storage is particularly attractive for applications where space and weight are critical constraints.
However, achieving and maintaining solid hydrogen storage presents formidable technical challenges. Efficient thermal insulation, controlled sublimation, and safe handling are all crucial considerations that demand innovative engineering solutions.
Hydrogen Isotopes in Nuclear Fusion
Cryogenics also plays a crucial role in the utilization of hydrogen isotopes, deuterium and tritium, in nuclear fusion reactors.
These isotopes, when heated to extremely high temperatures, can undergo nuclear fusion reactions, releasing vast amounts of energy. Cryogenic techniques are essential for separating, purifying, and handling these isotopes, as well as for cooling the superconducting magnets used to confine the fusion plasma.
The pursuit of nuclear fusion as a clean and sustainable energy source relies heavily on advancements in cryogenic technology.
Safety First: Experimental Considerations for Handling Cryogenic Hydrogen
Having examined the factors influencing the freezing point of hydrogen, it is now crucial to understand the broader scientific domain that enables its study and utilization: cryogenics. This field is not merely a passive observer; it is an active enabler, providing the tools and techniques necessary to explore matter at extremely low temperatures. However, with the immense potential of cryogenic hydrogen comes an equally significant responsibility to ensure safety. This section will delve into the crucial experimental considerations and safety protocols that are paramount when handling this volatile substance.
Essential Safety Equipment: A First Line of Defense
The safe manipulation of cryogenic hydrogen hinges on the use of specialized equipment designed to mitigate the inherent risks associated with ultra-low temperatures and hydrogen's flammability. Understanding the function and proper use of this equipment is paramount for anyone working in this field.
Cryogenic gloves are indispensable, designed to protect the hands from extreme cold that can cause severe burns. These gloves are typically multi-layered and made from materials with low thermal conductivity.
Eye protection, in the form of a face shield or goggles, is crucial for shielding the eyes from potential splashes of liquid hydrogen or fragments resulting from equipment failure.
Dewar flasks, specialized containers designed to minimize heat transfer, are used for storing and transporting liquid hydrogen. They are constructed with a double-walled design and a vacuum between the walls.
Pressure relief valves are critical safety devices installed on cryogenic systems to prevent over-pressurization, which can lead to catastrophic failures. These valves are meticulously calibrated to release excess pressure at a predetermined threshold.
Gas detectors play a vital role in monitoring hydrogen concentrations in the air. Hydrogen is odorless and colorless, making leaks difficult to detect without specialized equipment.
Personal Protective Equipment (PPE) extends beyond gloves and eye protection to include specialized clothing designed to minimize exposure to cryogenic fluids and potential hazards.
Flame-resistant clothing is crucial due to hydrogen's highly flammable nature. These garments are designed to resist ignition and provide a crucial layer of protection.
Specialized piping and fittings are essential for safely transferring cryogenic hydrogen. These components are designed to withstand extremely low temperatures and prevent leaks.
Necessary Safety Procedures: Minimizing Risk, Maximizing Safety
Beyond specialized equipment, adherence to rigorous safety procedures is equally critical when working with cryogenic hydrogen. These procedures are designed to minimize the risk of accidents and ensure a safe experimental environment for all personnel.
The importance of emergency procedures cannot be overstated. All personnel must be thoroughly trained in emergency protocols, including procedures for leak detection, fire suppression, and first aid. Regular drills are essential to ensure preparedness.
Ventilation is paramount to prevent the accumulation of hydrogen gas, which can lead to explosive conditions. Work areas must be equipped with adequate ventilation systems to ensure that hydrogen concentrations remain below flammable limits. Continuous monitoring of air quality is often necessary.
In conclusion, handling cryogenic hydrogen demands a comprehensive approach to safety, encompassing both specialized equipment and rigorous adherence to established protocols. Only through meticulous planning, thorough training, and a constant awareness of potential hazards can researchers and engineers safely unlock the vast potential of this remarkable element.
Materials Science and Engineering at Cryogenic Temperatures
Having examined the factors influencing the freezing point of hydrogen, it is now crucial to understand the broader scientific domain that enables its study and utilization: cryogenics. This field is not merely a passive observer; it is an active enabler, providing the tools and techniques necessary to manipulate matter at extreme temperatures, and, crucially, dictating the materials we can use to contain it. This section delves into the critical intersection of materials science and cryogenic engineering, specifically focusing on the challenges and considerations inherent in handling hydrogen at its lowest temperatures.
Materials Selection for Cryogenic Hydrogen Containment
The selection of appropriate materials for containing cryogenic hydrogen is not a trivial matter. At these extreme temperatures, ordinary materials can exhibit drastically altered properties, rendering them unsuitable or even dangerous for service.
Embrittlement, for instance, is a significant concern. Many metals, including commonly used steels, become brittle and prone to fracture at cryogenic temperatures. This phenomenon significantly limits their applicability in liquid hydrogen storage and transfer systems.
Austenitic stainless steels, such as 304 and 316, are often favored due to their retained ductility and toughness at cryogenic temperatures. Aluminum alloys also exhibit good performance in cryogenic environments due to their face-centered cubic (FCC) crystal structure.
However, even these materials require careful consideration. Factors such as weldability, fatigue resistance, and compatibility with hydrogen must be thoroughly evaluated. Hydrogen embrittlement, a phenomenon where hydrogen atoms diffuse into the metal lattice and reduce its ductility, can still pose a risk, particularly in high-pressure hydrogen systems.
Polymers also find niche applications in cryogenic systems, often as seals or insulation. However, their mechanical properties are also temperature-dependent, and careful selection is required to ensure they remain functional and leak-tight at cryogenic temperatures.
Critical Material Properties at Extremely Low Temperatures
The extreme cold profoundly impacts several critical material properties, necessitating a thorough understanding of their behavior at cryogenic conditions.
-
Thermal Conductivity: The ability of a material to conduct heat changes significantly at cryogenic temperatures. This has direct implications for insulation design, as the goal is to minimize heat leak into the cryogenic system.
-
Thermal Expansion: Materials contract upon cooling, and the degree of contraction varies depending on the material. Differential thermal contraction between dissimilar materials can induce stresses and lead to failure, necessitating careful design and material selection.
-
Strength and Ductility: As previously mentioned, many materials become stronger but more brittle at cryogenic temperatures. Maintaining adequate ductility to prevent catastrophic failure is paramount.
Application of Liquid Hydrogen in Aircraft Propulsion
The allure of liquid hydrogen as an aviation fuel is undeniable. Its high energy density-to-weight ratio offers the potential for significantly increased range and payload capacity compared to conventional jet fuel.
Moreover, hydrogen combustion produces only water as a byproduct, making it an attractive option for reducing greenhouse gas emissions from air travel.
However, realizing the potential of hydrogen-powered aircraft requires overcoming significant engineering challenges, particularly in the area of fuel storage and delivery.
Engineering Challenges in Aerospace Cryogenic Fuel Systems
The integration of cryogenic fuel systems into aircraft presents a unique set of engineering hurdles.
-
Tank Design and Insulation: Liquid hydrogen must be stored in highly insulated tanks to minimize boil-off, the evaporation of the fuel due to heat leak. Vacuum-jacketed tanks with multilayer insulation are commonly used, but these are bulky and add weight to the aircraft.
-
Fuel Delivery and Management: The fuel system must deliver hydrogen to the engines at the required pressure and flow rate, while also managing boil-off gas and preventing ice formation.
-
Material Compatibility: All materials in contact with liquid hydrogen must be compatible with the cryogenic environment and resistant to hydrogen embrittlement.
-
Safety: Ensuring the safe handling and storage of liquid hydrogen on board an aircraft is of paramount importance. Robust safety systems are needed to mitigate the risks of leaks, spills, and explosions.
Overcoming these challenges requires significant advancements in materials science, cryogenic engineering, and aircraft design. While hydrogen-powered aircraft are not yet commonplace, ongoing research and development efforts are paving the way for a future where hydrogen plays a significant role in sustainable aviation.
Organizational Involvement: Key Players in Cryogenic Research
Having explored the materials science and engineering challenges inherent in utilizing cryogenic hydrogen, it is essential to recognize the pivotal roles played by various organizations in advancing cryogenic research and applications. These institutions not only drive innovation but also provide critical data and infrastructure that underpin the entire field.
Space Agencies and Liquid Hydrogen Propulsion
Space exploration has long relied on the exceptional energy density of liquid hydrogen as a rocket propellant. NASA, the National Aeronautics and Space Administration, has been a pioneering force in utilizing liquid hydrogen in iconic programs like the Apollo missions and the Space Shuttle. The Space Shuttle's main engines, for example, were fueled by liquid hydrogen and liquid oxygen, delivering the immense thrust required to reach orbit.
Similarly, the European Space Agency (ESA) has incorporated liquid hydrogen technology in its Ariane rocket family. These rockets have been instrumental in launching numerous satellites and scientific payloads, demonstrating the continued importance of liquid hydrogen in modern spaceflight. The ongoing development of new launch systems further underscores the enduring role of cryogenic propellants.
NIST: A Cornerstone of Cryogenic Data
Beyond the application-driven endeavors of space agencies, organizations like the National Institute of Standards and Technology (NIST) play a vital role in providing the fundamental cryogenic property data essential for scientific and engineering progress. NIST's Physical Measurement Laboratory maintains extensive databases and conducts research to precisely characterize the behavior of materials at cryogenic temperatures.
This data is absolutely critical for designing safe and efficient cryogenic systems. Accurate knowledge of properties like thermal conductivity, heat capacity, and density is paramount for predicting system performance and preventing failures.
The Importance of Standardized Data
NIST's contributions extend beyond simply measuring properties. The institute also develops and maintains standardized measurement techniques, ensuring that data obtained from different laboratories can be reliably compared and used in collaborative research efforts. This standardization fosters confidence and accelerates progress in the field.
The availability of reliable cryogenic property data from NIST significantly reduces the risk and uncertainty associated with developing new cryogenic technologies, making it an indispensable resource for researchers and engineers worldwide. The data they provide is a crucial backbone to the entire field.
Video: Freezing Point of Hydrogen: Uses & Safety Guide
FAQs: Freezing Point of Hydrogen
Why is the freezing point of hydrogen important for its uses?
Understanding the freezing point of hydrogen is crucial because it dictates the temperature range where hydrogen remains a liquid. Many industrial and scientific applications require liquid hydrogen, so maintaining temperatures below its freezing point is essential for storage, transport, and utilization.
What safety precautions are needed when working with liquid hydrogen near its freezing point?
Safety requires specialized equipment and procedures due to hydrogen's extreme cold and flammability. Materials must be compatible with cryogenic temperatures to prevent embrittlement. Ventilation is vital to avoid hydrogen accumulation, and spark-proof tools are necessary to eliminate ignition sources.
What happens to hydrogen at its freezing point?
At its freezing point, hydrogen transitions from a liquid state to a solid. This phase change affects its density and volume, which has implications for storage tank design and pressure management. Controlling this transition requires precise temperature regulation.
How does the freezing point of hydrogen compare to other common cryogens?
The freezing point of hydrogen is significantly lower than many other common cryogens like liquid nitrogen or liquid helium. This extremely low temperature presents unique challenges for handling and storage, demanding specialized insulation and refrigeration techniques not needed for less extreme cryogenic fluids.
So, there you have it! Everything you need to know about the freezing point of hydrogen and its uses. While it's a fascinating and powerful element, remember to always prioritize safety when handling it. Stay informed, stay safe, and keep exploring the wonders of science!