Label Enzyme Diagram: Read & Understand It Now!
Enzyme diagrams, essential tools in biochemistry, offer a visual representation of enzyme-catalyzed reactions, which are vital to understanding metabolic pathways. Scientists at the European Bioinformatics Institute (EMBL-EBI) widely use and curate these diagrams to understand complex biological processes. The Michaelis-Menten kinetics model, a cornerstone in enzyme kinetics, provides a mathematical framework for analyzing reaction rates depicted in a label enzyme diagram. Accurate interpretation of the label enzyme diagram requires a thorough understanding of enzyme mechanisms; this knowledge is often enhanced through resources like KEGG (Kyoto Encyclopedia of Genes and Genomes), which provides comprehensive pathway information. Researchers like Leonor Michaelis have significantly contributed to our understanding of enzyme kinetics.
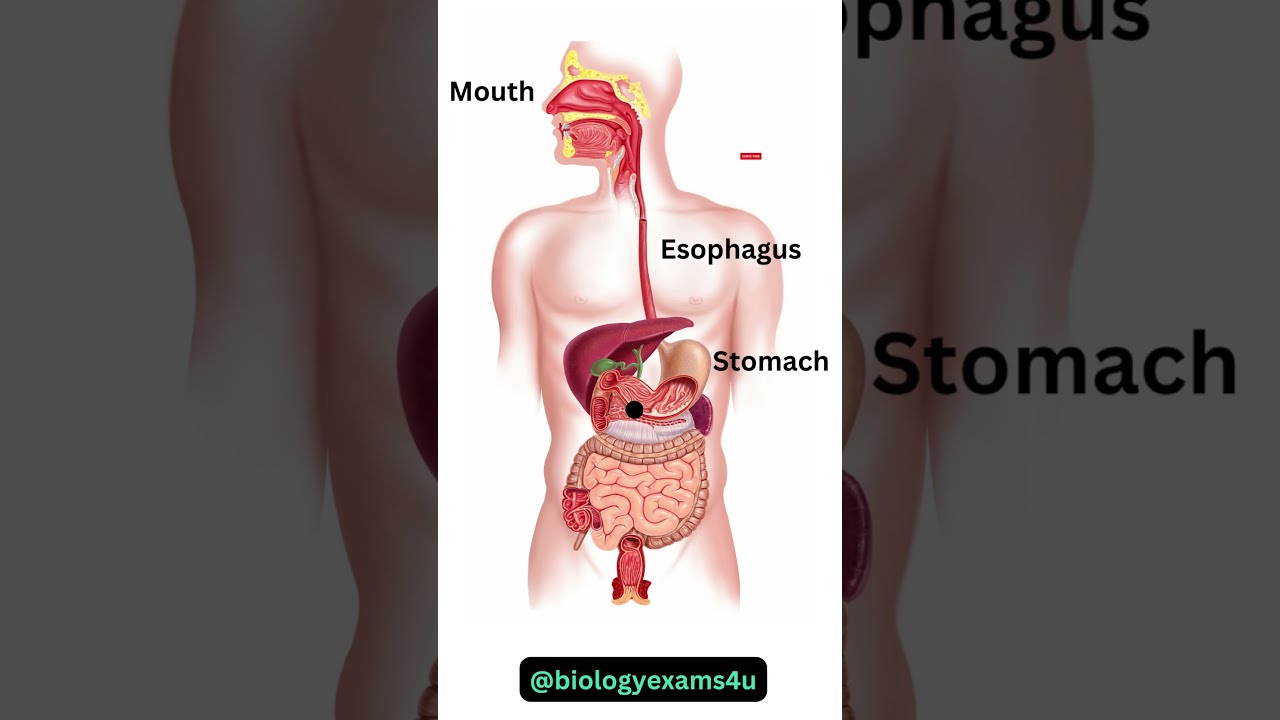
Image taken from the YouTube channel biologyexams4u , from the video titled Digestive System Animation || How Food moves through the Digestive System? .
Enzymes: The Unsung Heroes of Life
Enzymes are the workhorses of the biological world, acting as catalysts to accelerate the myriad chemical reactions essential for life. Without enzymes, these reactions would proceed far too slowly to sustain living organisms. They are, quite simply, indispensable.
The Vital Role of Enzymes
Enzymes are critical for a vast array of biological processes. Think of digestion, where enzymes break down complex food molecules into smaller, absorbable units.
Consider metabolism, where enzymes orchestrate the intricate pathways that provide energy and building blocks for cells. From muscle contraction to nerve function, enzymes are at the heart of it all.
Decoding the Language of Enzymes
To truly grasp the power of enzymes, it's crucial to understand the key players involved in their function. Let's dissect some essential terminology:
Enzyme: The Master Catalyst
The enzyme itself is the protein molecule that accelerates a specific biochemical reaction. Its unique three-dimensional structure is paramount to its function, allowing it to bind to specific molecules and facilitate their transformation.
Substrate: The Molecule of Interest
The substrate is the specific molecule upon which the enzyme acts. It is the starting material that the enzyme converts into a product.
Active Site: The Binding Hub
The active site is the region within the enzyme where the substrate binds. This site possesses a unique shape and chemical environment that perfectly complements the substrate, allowing for a highly specific interaction.
Enzyme-Substrate Complex: The Transient Duo
The enzyme-substrate complex is the temporary association formed when the enzyme binds to its substrate. This interaction is crucial for lowering the activation energy of the reaction and facilitating the conversion of the substrate into product.
Product: The End Result
The product is the molecule or molecules that result from the enzyme-catalyzed reaction. It represents the transformed substrate after the enzyme has done its work.
Cofactor: The Helper Molecule
A cofactor is a non-protein chemical compound that is required for the protein's biological activity. Cofactors can be metal ions or organic molecules.
Coenzyme: The Organic Assistant
A coenzyme is an organic non-protein cofactor that participates directly in the reaction, often carrying chemical groups or electrons. Many vitamins serve as coenzymes.
Inhibitor: The Activity Regulator
An inhibitor is a molecule that reduces the activity of an enzyme. Inhibitors can be competitive, binding to the active site, or non-competitive, binding elsewhere on the enzyme and altering its shape.
Activator: The Performance Enhancer
An activator is a molecule that increases the activity of an enzyme. Activators can enhance substrate binding or increase the catalytic efficiency of the enzyme.
Allosteric Site: The Regulatory Zone
The allosteric site is a location on the enzyme, distinct from the active site, that binds regulatory molecules. Binding at the allosteric site can induce conformational changes in the enzyme, affecting its activity.
Unlocking Enzyme Structure and Function: A Detailed Look
Having established the foundational terminology, we now turn our attention to the intricate relationship between enzyme structure and its remarkable function. The efficiency and specificity of an enzyme are intrinsically linked to its three-dimensional architecture and the dynamics of its interactions with substrates.
The Intricate Architecture of Enzymes
Enzymes are not merely linear chains of amino acids; their biological activity hinges on their precise folding into complex 3D structures. This folding is dictated by a hierarchy of interactions, from primary amino acid sequences to secondary structures (alpha-helices and beta-sheets) and tertiary conformations shaped by hydrophobic and hydrophilic forces.
The quaternary structure becomes relevant when multiple polypeptide subunits combine to form a functional enzyme. This intricate architecture is crucial for maintaining the active site's shape and creating an environment conducive to catalysis.
The Active Site: A Molecular Crucible
The active site is a specific region within the enzyme where the substrate binds and the chemical reaction occurs. Its unique shape and chemical properties are perfectly complementary to the substrate, like a lock and key.
Amino acid residues within the active site are strategically positioned to interact with the substrate through various forces, including hydrogen bonding, electrostatic interactions, and van der Waals forces. This precise arrangement ensures that the substrate is correctly oriented for the reaction to proceed.
Allosteric Regulation: Fine-Tuning Enzyme Activity
Enzymes are not simply on/off switches. Their activity can be finely tuned through allosteric regulation. Allosteric enzymes possess regulatory sites (allosteric sites) distinct from the active site, where modulator molecules can bind.
Binding of a modulator can induce conformational changes that either enhance or inhibit the enzyme's activity. This regulatory mechanism is essential for maintaining metabolic homeostasis and responding to changing cellular conditions.
Substrate Binding: The Initial Dance
The initial interaction between an enzyme and its substrate is a dynamic process, leading to the formation of the enzyme-substrate complex. This complex is a transient intermediate where the substrate is poised for chemical transformation.
The traditional "lock-and-key" model, while helpful for initial understanding, is oversimplified.
The induced fit model provides a more accurate representation.
It posits that the enzyme undergoes a conformational change upon substrate binding, molding itself around the substrate to optimize interactions and stabilize the transition state. This dynamic interaction is critical for efficient catalysis.
Catalytic Mechanisms: Lowering the Energy Barrier
Enzymes accelerate reactions by lowering the activation energy – the energy required to reach the transition state. They achieve this by providing an alternative reaction pathway with a lower energy barrier.
Enzymes employ various catalytic strategies, including:
- Acid-base catalysis: Transferring protons to facilitate bond breaking or formation.
- Covalent catalysis: Forming a temporary covalent bond between the enzyme and the substrate.
- Metal ion catalysis: Using metal ions to stabilize charged intermediates or mediate redox reactions.
Transition State Stabilization is a key mechanism. Enzymes preferentially bind to and stabilize the transition state, effectively lowering the activation energy and accelerating the reaction.
Product Release: The Final Act
Once the reaction is complete, the product is released from the enzyme. This release regenerates the enzyme, allowing it to catalyze another reaction. The enzyme's ability to bind tightly to the substrate and then release the product efficiently is critical for its catalytic turnover.
The entire process, from substrate binding to product release, is a carefully orchestrated sequence of events dictated by the enzyme's structure and dynamics, making enzymes the truly remarkable and essential molecules they are.
Enzyme Kinetics: Measuring the Speed of Life
Having unlocked the structural secrets that govern enzyme function, we now transition to the realm of enzyme kinetics. This is where we quantify the speed at which these biological catalysts operate. Understanding enzyme kinetics is essential for comprehending how enzymes respond to changes in their environment and how their activity can be modulated. Enzyme kinetics provides a framework for analyzing and predicting the behavior of enzymatic reactions, offering crucial insights into metabolic pathways and drug development.
Enzyme kinetics is, at its core, the study of the rates of enzyme-catalyzed reactions. It aims to elucidate the mechanisms by which enzymes accelerate biochemical transformations. By meticulously measuring reaction rates under varying conditions, we can gain a deeper understanding of the factors that influence enzyme activity. This knowledge is vital for optimizing industrial processes, designing effective pharmaceuticals, and unraveling the complexities of cellular metabolism.
Michaelis-Menten Kinetics: A Cornerstone Model
The Michaelis-Menten model is a fundamental concept in enzyme kinetics. It describes the relationship between the initial velocity of an enzymatic reaction and the substrate concentration.
This model relies on several key assumptions:
-
The formation of an enzyme-substrate complex (ES) is a reversible process.
-
The breakdown of the ES complex into product and free enzyme is the rate-limiting step.
-
The concentration of the substrate is significantly higher than the concentration of the enzyme.
The Michaelis-Menten equation mathematically expresses this relationship:
v = (Vmax [S]) / (Km + [S])
Where:
- v is the initial reaction velocity
- Vmax is the maximum reaction velocity
- [S] is the substrate concentration
- Km is the Michaelis constant
The Significance of Km: Affinity for Substrate
The Michaelis constant (Km) is a crucial parameter derived from the Michaelis-Menten equation. It represents the substrate concentration at which the reaction rate is half of Vmax. Km is often used as an indicator of the enzyme's affinity for its substrate. A low Km value indicates a high affinity, meaning the enzyme can achieve half of its maximum velocity at a relatively low substrate concentration. Conversely, a high Km value suggests a lower affinity.
Vmax: The Limit of Catalytic Speed
The maximum velocity (Vmax) represents the theoretical upper limit of the reaction rate when the enzyme is fully saturated with substrate. It signifies the point at which every enzyme molecule is actively engaged in catalysis, and increasing the substrate concentration will not further accelerate the reaction. Vmax is directly proportional to the enzyme concentration and provides insights into the enzyme's catalytic efficiency.
Factors Affecting Enzyme Activity: Modulating the Speed
Enzyme activity is not static; it is influenced by a variety of environmental factors. Understanding these influences is crucial for controlling and optimizing enzymatic reactions.
The Impact of Temperature, pH, and Ionic Strength
-
Temperature can significantly impact enzyme activity. Generally, increasing the temperature increases the reaction rate up to a certain point. Beyond this optimal temperature, the enzyme can denature, leading to a loss of activity.
-
pH affects the ionization state of amino acid residues in the active site, which can alter substrate binding and catalytic activity. Each enzyme has an optimal pH range in which it functions most effectively.
-
Ionic strength can also influence enzyme activity by affecting the interactions between the enzyme and its substrate or cofactors.
Substrate Concentration: Driving the Reaction
As described by the Michaelis-Menten equation, substrate concentration plays a critical role in determining the rate of an enzymatic reaction. At low substrate concentrations, the reaction rate increases almost linearly with increasing substrate. However, as the substrate concentration increases, the rate eventually plateaus, approaching Vmax. This saturation effect reflects the limited number of available enzyme molecules to bind substrate.
Enzyme Inhibition: Regulation and Control
Having unlocked the structural secrets that govern enzyme function, we now transition to the realm of enzyme kinetics. This is where we quantify the speed at which these biological catalysts operate. Understanding enzyme kinetics is essential for comprehending how enzymes respond to changes in their environment and, crucially, how their activity can be modulated through inhibition.
Enzyme inhibition is a vital regulatory process, allowing cells to fine-tune metabolic pathways and respond to changing conditions. It is the control switch that turns on and off a particular activity.
Inhibition comes in several forms, each with distinct mechanisms and effects on enzyme kinetics. From directly blocking the active site to inducing conformational changes, enzyme inhibitors play a crucial role in maintaining cellular homeostasis. Understanding how these different types of inhibitors work provides insights into the sophisticated regulatory networks that govern life.
The Arsenal of Inhibition: Types of Enzyme Inhibition
Enzyme inhibition is not a one-size-fits-all phenomenon. There are several distinct types of enzyme inhibition, each with a unique mechanism of action and characteristic effect on enzyme kinetics. These inhibitors act in different manners.
Understanding the differences between these types of inhibition is crucial for comprehending how enzyme activity is regulated in biological systems.
Competitive Inhibition: A Direct Confrontation
Competitive inhibition is perhaps the most intuitive type of enzyme inhibition. In this scenario, the inhibitor molecule bears a striking resemblance to the substrate. The inhibitor directly competes with the substrate for binding to the enzyme's active site.
The result is a reduced rate of catalysis because the enzyme can't bind to the substrate. The inhibitor gets in the way, and thus preventing the enzyme from doing its job.
Kinetic Impact: Km and Vmax in Competitive Inhibition
The presence of a competitive inhibitor increases the apparent Km of the enzyme. That is, it requires a higher substrate concentration to achieve half of the maximum velocity. This is because the substrate must now "compete" with the inhibitor for binding.
However, the Vmax remains unchanged. Given a sufficiently high concentration of substrate, the substrate can outcompete the inhibitor, eventually achieving the maximum reaction rate.
Non-Competitive Inhibition: An Indirect Attack
Non-competitive inhibition takes a different approach. Instead of directly competing for the active site, the inhibitor binds to a different location on the enzyme. This binding induces a conformational change in the enzyme, distorting the active site and reducing its affinity for the substrate.
The result is a slower or inhibited reaction. The inhibitor distorts the enzyme structure, thus reducing enzyme activity.
Kinetic Impact: Km and Vmax in Non-Competitive Inhibition
In non-competitive inhibition, the Km remains unchanged. The inhibitor does not affect the enzyme's affinity for the substrate, as it binds to a different site.
However, the Vmax is reduced. Even at saturating substrate concentrations, the enzyme cannot achieve its maximum reaction rate because the inhibitor is interfering with its catalytic activity.
Uncompetitive Inhibition: A Subtle Tactic
Uncompetitive inhibition is a more subtle form of regulation. In this case, the inhibitor binds only to the enzyme-substrate complex, not to the free enzyme. This binding further distorts the active site, preventing the complex from proceeding to form products.
Uncompetitive inhibition is a little bit more hidden and complex. The inhibitor prevents the enzyme-substrate complex from doing its job.
Kinetic Impact: Km and Vmax in Uncompetitive Inhibition
Uncompetitive inhibition affects both Km and Vmax. The Km is decreased because the inhibitor essentially "traps" the enzyme-substrate complex, increasing the apparent affinity of the enzyme for the substrate.
The Vmax is also decreased because the inhibitor prevents the complex from proceeding to product formation, reducing the overall reaction rate.
Feedback Inhibition: A Regulatory Loop
Feedback inhibition is a powerful regulatory mechanism that allows cells to maintain homeostasis and prevent the overproduction of metabolic intermediates. This is done through a process of self-regulation.
In feedback inhibition, the end product of a metabolic pathway acts as an inhibitor of an earlier enzyme in the same pathway. This creates a negative feedback loop: as the concentration of the end product increases, it inhibits the enzyme, slowing down the production of more end product.
The allosteric site plays a critical role in feedback regulation. The end product typically binds to the allosteric site of the enzyme, inducing a conformational change that reduces its activity. This conformational change can decrease the enzyme's affinity for its substrate or reduce its catalytic efficiency.
This elegant mechanism ensures that the cell produces just the right amount of each metabolite, preventing waste and maintaining optimal metabolic balance.
Common Enzymatic Reactions: A Practical Overview
After unraveling the intricacies of enzyme inhibition, it's time to spotlight specific enzymatic reactions that are essential for life. Enzymes are not just theoretical concepts; they're the workhorses of biology, driving countless processes within our cells and ecosystems.
Let's explore some prominent examples to solidify our grasp of their indispensable contributions.
Hydrolysis: Water as a Molecular Scissor
Hydrolysis, meaning "to split with water," represents a cornerstone of enzymatic reactions. This process involves cleaving a chemical bond by adding a water molecule.
Enzymes known as hydrolases catalyze this vital reaction.
Digestive Hydrolysis
A prime example is the digestion of carbohydrates, proteins, and fats.
Amylases hydrolyze starch into simpler sugars. Proteases break down proteins into amino acids. Lipases cleave fats into glycerol and fatty acids.
These processes are fundamental for nutrient absorption and energy production.
Other Examples of Hydrolysis
Hydrolysis plays a vital role beyond digestion.
For instance, phosphatases hydrolyze phosphate ester bonds, a crucial step in signal transduction pathways. Nucleases hydrolyze phosphodiester bonds in nucleic acids, vital for DNA and RNA metabolism.
Phosphorylation: Adding Power with Phosphate
Phosphorylation, the addition of a phosphate group to a molecule, is another ubiquitous enzymatic reaction. Kinases are the enzymes responsible for catalyzing this process, often using ATP (adenosine triphosphate) as the phosphate donor.
The Significance of Phosphorylation
Phosphorylation acts as a molecular switch, modulating protein activity and initiating signaling cascades.
The addition of a negatively charged phosphate group can alter the protein's conformation. This can affect its ability to interact with other molecules, or its catalytic activity.
Examples of Phosphorylation
Many signaling pathways rely on phosphorylation.
The MAPK (mitogen-activated protein kinase) pathway, crucial for cell growth and differentiation, involves a series of phosphorylation events. Receptor tyrosine kinases (RTKs) are activated by phosphorylation, triggering intracellular signaling.
Dephosphorylation: Reversing the Switch
Dephosphorylation, the removal of a phosphate group, serves as the counterpart to phosphorylation. Phosphatases catalyze this reaction, reversing the effects of kinases.
The Importance of Dephosphorylation
Dephosphorylation is just as important as phosphorylation, allowing cells to fine-tune signaling pathways and prevent overstimulation.
Together, phosphorylation and dephosphorylation create a dynamic equilibrium that controls cellular processes.
Examples of Dephosphorylation
Protein phosphatases play crucial roles in regulating cell cycle progression, gene expression, and metabolism.
For example, the enzyme calcineurin dephosphorylates transcription factors, influencing immune responses and neuronal development.
Oxidation-Reduction (Redox) Reactions: Electron Transfer
Oxidation-reduction (redox) reactions involve the transfer of electrons between molecules. Oxidases and reductases are the enzymes that catalyze these reactions, which are essential for energy production and detoxification.
Redox Reactions in Cellular Respiration
Cellular respiration relies heavily on redox reactions to extract energy from glucose.
Electrons are passed from one molecule to another, ultimately driving the production of ATP. Enzymes like dehydrogenases play pivotal roles in these electron transfer chains.
Other Redox Reactions
Redox reactions are crucial in photosynthesis, where plants use light energy to convert carbon dioxide and water into glucose. They are also vital in detoxification processes, where enzymes like cytochrome P450s oxidize harmful substances, making them easier to eliminate from the body.
Visualizing Enzymes: Tools for Understanding and Presentation
After unraveling the intricacies of enzyme inhibition, it's time to spotlight specific enzymatic reactions that are essential for life. Enzymes are not just theoretical concepts; they're the workhorses of biology, driving countless processes within our cells and ecosystems.
Let's explore some prominent tools for visually understanding and presenting these concepts effectively.
The Power of Visual Communication in Enzymology
Understanding enzymes, their structures, and their mechanisms can be challenging. Textbooks and lectures often fall short in conveying the complex three-dimensional interactions that define enzyme function. This is where visual communication tools come into play.
Diagrams, illustrations, and animations can transform abstract concepts into tangible and memorable visual experiences.
These visuals are essential for not only learning about enzymes but also communicating their importance to broader audiences.
BioRender and Mind the Graph: Catalysts for Visual Creation
Several software platforms cater specifically to scientists and educators seeking to create professional-quality visuals. Two prominent players are BioRender and Mind the Graph.
Both offer extensive libraries of pre-designed icons, templates, and tools optimized for scientific communication.
BioRender: Streamlining Scientific Illustration
BioRender has become a go-to platform for researchers looking to create publication-ready figures. Its intuitive interface and vast library of biological icons make it easy to assemble complex diagrams quickly.
From enzyme structures to metabolic pathways, BioRender empowers users to create visually appealing and scientifically accurate representations.
Key features of BioRender include drag-and-drop functionality, customizable icons, and pre-designed templates for common biological processes.
Mind the Graph: Elevating Scientific Infographics
Mind the Graph focuses on creating visually engaging infographics and presentations. It caters to researchers who need to communicate complex scientific information to a broader audience.
Mind the Graph offers tools for creating visually stunning summaries of research findings. It also provides visually rich educational materials.
Mind the Graph excels in its ability to generate professional-looking infographics that explain complex concepts in a clear and accessible manner.
Practical Applications: Showcasing Enzymes in Action
Here are some examples of how BioRender and Mind the Graph can be used to illustrate enzyme-related concepts:
-
Enzyme Structure and Function: Create detailed diagrams of enzyme active sites, highlighting key amino acid residues involved in substrate binding and catalysis. Visualize the induced fit model showing how the enzyme changes shape upon substrate binding.
-
Enzyme Kinetics: Develop informative graphs illustrating Michaelis-Menten kinetics, showing the relationship between substrate concentration and reaction velocity. Visually represent the effects of competitive and non-competitive inhibitors on enzyme activity.
-
Metabolic Pathways: Construct comprehensive diagrams of metabolic pathways, highlighting the enzymes involved in each step. Show how feedback inhibition regulates these pathways.
-
Mechanism of Action: Animate the step-by-step mechanism of enzymatic reactions, illustrating the formation of transition states and the role of cofactors.
Enhancing Understanding and Engagement
By leveraging visual communication tools, educators and researchers can significantly enhance understanding and engagement with enzyme-related concepts.
Visuals break down complex information into digestible chunks. They also help audiences retain information more effectively. Furthermore, visually appealing diagrams can make presentations and publications more engaging and impactful.
Ultimately, these tools empower us to explore the fascinating world of enzymes in a more accessible and effective way.
Video: Label Enzyme Diagram: Read & Understand It Now!
FAQ: Understanding Enzyme Diagrams
What are the key components typically shown in a label enzyme diagram?
A label enzyme diagram usually depicts the enzyme, the substrate(s), the active site (where the substrate binds), and the product(s). It may also show cofactors or coenzymes if they're involved.
How does a label enzyme diagram illustrate enzyme specificity?
The diagram highlights the active site's shape, which is precisely complementary to the shape of the substrate. This illustrates how enzymes only bind and catalyze reactions with specific molecules, showcasing enzyme specificity. The label enzyme diagram will show how the substrate perfectly fits into the active site.
What does an arrow indicate in a label enzyme diagram?
An arrow typically represents the chemical reaction being catalyzed by the enzyme. It shows the transformation of the substrate(s) into the product(s). Look for the arrow to understand the reaction direction in a label enzyme diagram.
What is the significance of labeling the active site in a label enzyme diagram?
The active site labeling is crucial because it points out the region where the enzyme interacts with the substrate. It’s where the catalysis actually happens. Understanding the active site helps explain how the enzyme works, as depicted in the label enzyme diagram.
So, next time you're staring at a complex biochemical pathway and feeling lost, remember this guide to understanding the label enzyme diagram! Hopefully, you'll feel a little more confident navigating those diagrams and deciphering the amazing world of enzymes. Good luck, and happy studying!