Ball and Stick Model: A Beginner's Chemistry Guide
Embark on a thrilling journey into the microscopic world with the ball and stick model, a cornerstone in understanding molecular structures! Renowned chemist Linus Pauling significantly advanced our comprehension of chemical bonds, and the ball and stick model visually represents these bonds as connections between atoms. The model, a staple in chemistry education, is commonly utilized within academic chemistry departments to illustrate complex molecules. Software packages like ChemDraw also offer digital versions, allowing students to visualize and manipulate molecules in ways that enhance their learning experience.
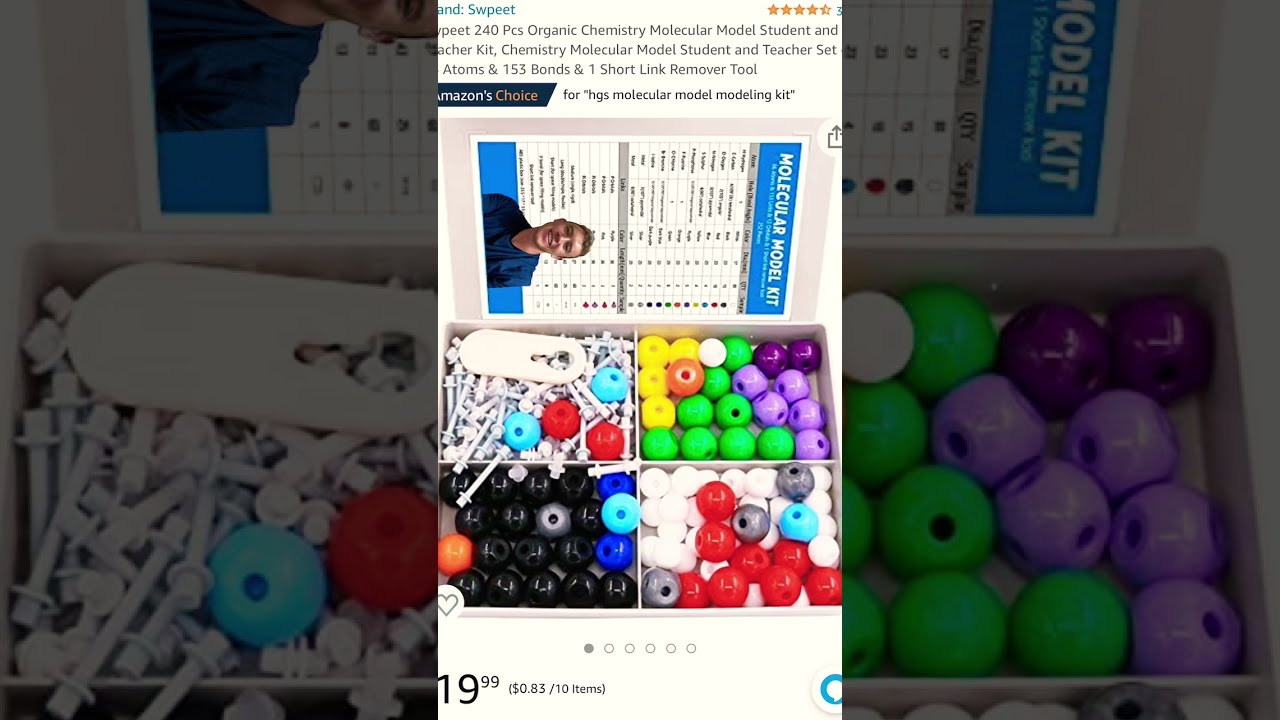
Image taken from the YouTube channel What I spend my paychecks on , from the video titled Ball and stick chemistry set #bestfinds #amazonfinds #shopping #chemistry .
Unlocking the Secrets of the Universe, One Molecule at a Time
Imagine holding the building blocks of life in your hands. What if you could visualize the intricate dance of atoms that determines everything from the color of a flower to the potency of a medicine?
This isn't science fiction; it's the power of molecular models.
They are not mere toys; they are portals to understanding the very fabric of reality.
The Significance of Molecular Structures
At the heart of chemistry lies the concept of molecular structure – the three-dimensional arrangement of atoms within a molecule. This seemingly simple arrangement dictates a molecule's properties, reactivity, and ultimately, its function.
Think of it like a finely tuned machine.
Change the position of one gear, and the whole mechanism can grind to a halt, or perform a completely different function. In the same way, altering a molecule's structure can dramatically impact its behavior.
Understanding molecular structures is therefore crucial for countless applications.
It's the key to:
- Designing new drugs.
- Developing advanced materials.
- Unraveling the mysteries of biological processes.
- Predicting chemical reactions.
Molecular Models: Essential Tools for Discovery
So, how do we unlock these molecular secrets?
This is where molecular models come into play. These tangible representations of molecules allow us to visualize the invisible, to grasp the complex shapes and interactions that govern the molecular world.
Whether they're simple ball-and-stick models or sophisticated computer simulations, molecular models provide an invaluable tool for both scientists and students.
They allow us to:
- Translate abstract concepts into concrete forms.
- Predict molecular behavior.
- Develop intuitive understanding.
- Foster innovation in countless fields.
The Foundation: Core Molecular Concepts
Unlocking the Secrets of the Universe, One Molecule at a Time Imagine holding the building blocks of life in your hands. What if you could visualize the intricate dance of atoms that determines everything from the color of a flower to the potency of a medicine? This isn't science fiction; it's the power of molecular models. They are not mere toys; they are windows into a world unseen, tools that empower us to grasp the fundamental principles of chemistry. But to truly appreciate the insights they offer, we must first lay a solid foundation of core molecular concepts. So, let’s dive in and build that foundation together!
Molecular Structure: The Blueprint of a Molecule
Think of molecular structure as the blueprint of a molecule. It describes the arrangement of atoms within the molecule, specifying which atoms are bonded to which. This arrangement is not arbitrary; it dictates the molecule's properties and behavior. A slight change in structure can dramatically alter a compound's reactivity, color, or even its biological activity.
Consider the simple example of ethanol and dimethyl ether. Both have the same molecular formula (C2H6O), but their differing atomic arrangements give them drastically different properties. Ethanol is the alcohol in alcoholic beverages, while dimethyl ether is a gas used as an aerosol propellant. This difference arises solely from the way the atoms are connected, emphasizing the crucial role of molecular structure.
Molecular Geometry: Shape Matters
While molecular structure focuses on connectivity, molecular geometry delves into the three-dimensional arrangement of atoms in space. It's the molecule's actual shape. Importantly, molecular geometry differs from electronic geometry, which considers the arrangement of all electron pairs (both bonding and lone pairs) around a central atom.
Molecular geometry is crucial because it influences how a molecule interacts with other molecules. Imagine trying to fit two puzzle pieces together – their shapes must complement each other. Similarly, the shape of a molecule determines how it binds to receptors, interacts with enzymes, or packs into a crystal lattice. Understanding molecular geometry allows us to predict and explain a wide range of chemical and biological phenomena.
Chemical Bonds: The Glue That Holds Molecules Together
Chemical bonds are the attractive forces that hold atoms together to form molecules. These forces arise from the interactions of electrons and nuclei. The type of chemical bond significantly affects the properties of the resulting molecule.
Types of Chemical Bonds
-
Covalent Bonds: Formed by the sharing of electrons between atoms. These are common in organic molecules and contribute to strong, stable structures.
-
Ionic Bonds: Result from the transfer of electrons from one atom to another, creating ions with opposite charges that attract each other. Think of sodium chloride (table salt) as a prime example.
-
Metallic Bonds: Found in metals, where electrons are delocalized and shared among a lattice of atoms, leading to properties like high conductivity and malleability.
Atoms: The Elemental Building Blocks
Atoms are the fundamental building blocks of matter. Each element on the periodic table represents a unique type of atom, characterized by its number of protons. The properties of an atom, such as its electronegativity and size, influence the types of bonds it can form and the overall structure of the molecules it participates in. Different combinations of atoms lead to the incredible diversity of molecules found in nature and synthesized in laboratories.
Molecules: A Symphony of Atoms
Molecules are formed when two or more atoms are held together by chemical bonds. From the simplest diatomic molecules like oxygen (O2) to complex biomolecules like proteins and DNA, molecules are the foundation of all matter. Their diversity is staggering, and their importance cannot be overstated.
Molecules participate in countless processes, from the biochemical reactions that sustain life to the industrial processes that produce the materials we use every day. Understanding their structure, geometry, and bonding is essential for comprehending the world around us. With a firm grasp of these core concepts, we are ready to unlock the secrets encoded within molecular models and delve deeper into the fascinating world of chemistry.
Predicting and Visualizing Molecular Shapes with VSEPR Theory
With a firm grasp of fundamental molecular concepts, we can now explore the fascinating world of predicting and visualizing the shapes of molecules. This is where the Valence Shell Electron Pair Repulsion (VSEPR) theory comes into play, offering a powerful framework for understanding how electron pairs around a central atom influence the overall molecular geometry.
VSEPR Theory: Unveiling Molecular Architecture
VSEPR theory is based on the simple yet profound idea that electron pairs, whether in bonds or lone pairs, repel each other.
This repulsion forces them to arrange themselves as far apart as possible in three-dimensional space, thus dictating the molecule's shape. It's like balloons tied together; they naturally push each other away, resulting in specific arrangements!
Applying VSEPR: A Step-by-Step Approach
Using VSEPR involves a few key steps:
-
Draw the Lewis structure of the molecule. This provides the connectivity of atoms and shows all bonding and non-bonding electron pairs.
-
Count the number of electron groups around the central atom. An electron group can be a single bond, a double bond, a triple bond, or a lone pair. Each counts as one group.
-
Determine the electron geometry. This is the arrangement of all electron groups around the central atom. Common electron geometries include linear, trigonal planar, tetrahedral, trigonal bipyramidal, and octahedral.
-
Determine the molecular geometry. This is the arrangement of only the atoms in the molecule. Lone pairs influence the electron geometry, but they are not "visible" in the molecular geometry. This can lead to shapes like bent or pyramidal even with a tetrahedral electron geometry.
Common Molecular Shapes: A Visual Tour
-
Linear: Molecules with two electron groups arranged 180° apart, like carbon dioxide (CO2), adopt a linear shape.
-
Trigonal Planar: With three electron groups, molecules like boron trifluoride (BF3) arrange themselves in a flat, triangular shape with bond angles of 120°.
-
Bent: Molecules with a trigonal planar electron geometry but one lone pair, such as sulfur dioxide (SO2), adopt a bent shape. The lone pair repels the bonding pairs more strongly, squeezing the bond angle slightly.
-
Tetrahedral: Four electron groups, as in methane (CH4), result in a tetrahedral shape with bond angles of approximately 109.5°.
-
Pyramidal: Molecules with a tetrahedral electron geometry but one lone pair, such as ammonia (NH3), adopt a pyramidal shape.
Bond Length and Bond Angle: Defining Molecular Dimensions
Beyond the overall shape, bond length and bond angle are critical parameters that define a molecule's precise dimensions.
Bond Length: The Distance Between Atoms
Bond length is the average distance between the nuclei of two bonded atoms.
It's influenced by factors like the size of the atoms and the bond order (single, double, or triple). Shorter bond lengths generally indicate stronger bonds.
Bond Angle: The Shape's Blueprint
Bond angle is the angle formed between three atoms in a molecule.
It directly contributes to the molecule's overall shape and affects properties like polarity and reactivity.
Space-Filling Models: Visualizing Molecular Volume
While VSEPR theory helps us predict shapes, space-filling models offer a tangible way to visualize the space occupied by atoms in a molecule.
These models represent atoms as spheres with radii proportional to their van der Waals radii, providing a sense of the molecule's overall size and shape.
They're especially useful for understanding how molecules pack together in solids and liquids, and how they might interact with other molecules.
Understanding molecular shape is fundamental to understanding molecular behavior. VSEPR theory, alongside the visualization of molecular properties via bond lengths, angles, and space-filling models, provides an indispensable toolset for chemists and students alike!
Representing Molecular Structures: From 2D to Simplified
With a firm grasp of fundamental molecular concepts, we can now explore the different ways chemists represent molecular structures, moving beyond simple definitions to visual tools that aid in understanding molecular architecture. These representations, ranging from detailed structural formulas to streamlined skeletal notations, each offer unique insights into a molecule's composition and connectivity, serving as indispensable tools in chemical communication and analysis.
Structural Formulas: Detailing Connectivity in Two Dimensions
Structural formulas represent molecules in two dimensions, explicitly showing each atom and the bonds connecting them. They excel at illustrating the connectivity between atoms, clearly indicating which atom is bonded to which.
Consider ethanol (C₂H₅OH). Its structural formula reveals not just the types and numbers of atoms, but also how they are arranged: two carbon atoms linked together, one carbon bonded to three hydrogen atoms, the other bonded to two hydrogens and an -OH group.
This level of detail can be incredibly useful for understanding reaction mechanisms or identifying functional groups within a molecule.
However, structural formulas have limitations. They don't accurately portray the three-dimensional shape of a molecule. They also become cumbersome for larger, more complex molecules.
Skeletal Formulas: Embracing Simplicity
Skeletal formulas, also known as line-angle formulas, offer a streamlined approach, particularly popular in organic chemistry. These diagrams drastically simplify the representation of molecules by employing a clever convention: carbon atoms are implied at the corners and ends of lines, and hydrogen atoms bonded to carbon are not explicitly drawn.
This makes skeletal formulas incredibly efficient for representing complex organic structures, allowing chemists to focus on the essential features of the molecule, such as functional groups, rings, and stereocenters, without being overwhelmed by a clutter of C's and H's.
Decoding the Skeletal Structure
Understanding skeletal formulas requires a bit of "decoding." Each line represents a bond. An intersection of two lines or the end of a line signifies a carbon atom.
We assume that each carbon atom has enough hydrogen atoms attached to satisfy its tetravalency (ability to form four bonds), unless other atoms are explicitly shown.
For example, cyclohexane (C₆H₁₂) is represented as a simple hexagon. Each corner of the hexagon represents a carbon atom, and each carbon is implicitly bonded to two hydrogen atoms.
Advantages and Best Use Cases
The elegance of skeletal formulas lies in their simplicity and clarity. They allow for rapid drawing and interpretation of organic molecules, making them indispensable for quickly conveying structural information.
They are particularly useful for:
- Representing cyclic structures.
- Highlighting functional groups.
- Depicting stereochemistry.
Skeletal formulas are not without limitations. For beginners, the implicit nature of carbon and hydrogen atoms can be initially confusing.
However, with practice, they become second nature and an essential tool in any chemist's toolkit.
Advanced Concepts in Molecular Modeling: Isomers and Stereochemistry
Representing Molecular Structures: From 2D to Simplified. With a firm grasp of fundamental molecular concepts, we can now explore the different ways chemists represent molecular structures, moving beyond simple definitions to visual tools that aid in understanding molecular architecture. These representations, ranging from detailed structural formulas to simplified skeletal notations, provide essential insights into a molecule's composition and connectivity. Building on this foundation, we now venture into even more complex realms of molecular architecture: isomerism and stereochemistry.
This opens doors to understanding the nuances of molecular behavior and the crucial role these subtle differences play in the properties and functions of chemical compounds. So, let's dive in!
Unveiling Isomers: Same Formula, Different Structures
Isomers are molecules that share the same molecular formula but possess distinct structural arrangements.
This seemingly small difference can lead to drastically different physical and chemical properties. Understanding isomers is vital, as they are abundant in organic chemistry and biochemistry.
Structural Isomers: Different Connectivity
Structural isomers, also known as constitutional isomers, are molecules that differ in the way their atoms are connected.
Think of it as rearranging the order in which you link the building blocks of a molecule. For example, butane and isobutane both have the formula C₄H₁₀.
However, butane features a straight chain of four carbon atoms, while isobutane has a branched structure. This seemingly minor change affects the molecule's boiling point, reactivity, and other key properties.
Stereoisomers: Same Connectivity, Different Spatial Arrangement
Stereoisomers, in contrast to structural isomers, have the same atomic connectivity but differ in the spatial arrangement of their atoms.
This introduces a new level of complexity, as the 3D arrangement of atoms can profoundly impact how a molecule interacts with its environment.
There are two primary types of stereoisomers: enantiomers and diastereomers.
Enantiomers: Mirror Images That Aren't Superimposable
Enantiomers are stereoisomers that are non-superimposable mirror images of each other, much like your left and right hands.
This "handedness," known as chirality, is a critical concept in stereochemistry.
Enantiomers often exhibit identical physical properties, such as melting point and boiling point. However, they interact differently with polarized light and can have dramatically different effects in biological systems.
Diastereomers: Stereoisomers That Aren't Mirror Images
Diastereomers are stereoisomers that are not mirror images of each other.
They have different physical properties, such as melting points and boiling points, and their chemical reactivity can also vary significantly.
Cis-trans isomers (also known as geometric isomers), which arise from restricted rotation around a double bond or in cyclic systems, are a classic example of diastereomers.
Stereochemistry: The Importance of 3D Arrangement
Stereochemistry is the study of the three-dimensional arrangement of atoms within molecules and how this arrangement affects their chemical and physical properties. It's a field with profound implications, especially in drug design and biological systems.
Stereochemistry in Drug Design: A Matter of Fit
In drug design, stereochemistry is paramount.
Many biological molecules, such as enzymes and receptors, are chiral. This means they interact differently with different enantiomers of a drug molecule.
One enantiomer might fit perfectly into the active site of an enzyme, acting as a potent therapeutic agent.
The other enantiomer, however, might not fit at all, or worse, it could bind to a different site and cause unwanted side effects.
The infamous case of thalidomide serves as a stark reminder of the importance of stereochemistry in drug development. One enantiomer of thalidomide was an effective treatment for morning sickness, while the other caused severe birth defects.
Stereochemistry in Biological Systems: Nature's Handedness
Stereochemistry plays a vital role in biological systems. Many biological molecules, such as amino acids and sugars, are chiral. Enzymes, the catalysts of biological reactions, are highly stereospecific. They can distinguish between different stereoisomers and catalyze reactions with only one specific form.
This stereospecificity is essential for the proper functioning of biological systems.
Molecular models help us visualize and understand these complex 3D arrangements, enabling us to design better drugs, understand biological processes, and unravel the intricacies of the molecular world.
The Intersection of Disciplines and Craft: Fields of Study and Materials in Molecular Modeling
From visualizing complex stereoisomers to understanding the nuances of molecular geometry, molecular models wouldn’t exist without a convergence of scientific understanding and material craftsmanship. Let’s enthusiastically delve into the key disciplines that shape our comprehension of molecular structures and the tangible components used to bring these abstract concepts to life.
Core Scientific Disciplines
Several scientific fields contribute directly to the creation, interpretation, and application of molecular models. These fields provide the foundational knowledge that makes accurate and insightful modeling possible.
Organic Chemistry: The Carbon-Based Universe
At the heart of molecular modeling lies organic chemistry—the systematic study of carbon-containing compounds. Given carbon's unique ability to form stable chains and rings, organic chemistry explores a vast array of molecules crucial to life and industry.
Understanding organic chemistry is essential for building accurate models that reflect real-world molecules, predicting their behavior, and designing new compounds with specific properties. It is, in essence, the backbone upon which our molecular understanding is built.
Other Relevant Fields
While organic chemistry often takes center stage, other fields like inorganic chemistry, physical chemistry, and biochemistry also play vital roles. Each discipline provides a unique lens through which to understand molecular behavior.
The Material Palette: Constructing Molecular Realities
Creating physical molecular models requires a careful selection of materials that balance durability, accuracy, and ease of use. The choice of materials impacts not only the model's appearance but also its functionality and educational value.
Plastic: Versatility in Form and Function
Plastic is a widely used material in modern molecular models due to its versatility and cost-effectiveness. It can be easily molded into various shapes and sizes, allowing for precise representation of atoms and bonds.
Different types of plastics offer varying degrees of flexibility and rigidity, catering to different modeling needs. The vibrant colors available with plastic also aid in visually distinguishing different atoms and functional groups.
Metal: Stability and Connectivity
Metal components, particularly metal connectors or "sticks," provide structural integrity to molecular models. These connectors link plastic or other atom representations, ensuring the model retains its shape.
The rigidity of metal connectors helps demonstrate the fixed angles and lengths of chemical bonds, an important aspect of molecular geometry. Metal's durability also makes it suitable for models intended for frequent use.
Paint and Coloring Agents: Distinguishing the Players
Visual clarity is paramount in molecular models, and paint or coloring agents play a critical role in differentiating atoms. A standardized color scheme, such as the CPK coloring scheme, is often employed to represent different elements.
For example, carbon atoms are typically black, oxygen atoms are red, and hydrogen atoms are white. Consistent color-coding makes it easier to identify elements and visualize the overall molecular structure at a glance.
By combining the principles of organic chemistry with the practical application of materials like plastic, metal, and coloring agents, molecular modeling becomes a powerful tool for education, research, and innovation. The intersection of these disciplines ensures that models are not only visually appealing but also scientifically accurate and insightful.
Video: Ball and Stick Model: A Beginner's Chemistry Guide
FAQs
What do the balls and sticks represent in a ball and stick model?
In a ball and stick model, the balls represent atoms, and the sticks represent the chemical bonds between those atoms. Different colored balls are often used to indicate different elements like carbon, hydrogen, or oxygen.
What information isn't shown by a ball and stick model?
A ball and stick model doesn't accurately represent the true size of atoms or the actual length and strength of chemical bonds. It simplifies the structure and doesn't show electron distribution or the three-dimensional shape beyond the basic connectivity.
Why is the ball and stick model useful for beginners?
The ball and stick model provides a visually simple way to understand the arrangement of atoms in a molecule. It helps beginners grasp basic concepts like molecular structure, bonding patterns, and spatial relationships between atoms without needing advanced knowledge.
How does a ball and stick model compare to other molecular models?
Compared to space-filling models, the ball and stick model makes it easier to see the connections and structure within the molecule, rather than only its overall shape. While less realistic than space-filling models, it is simpler to visualize and understand for learning molecular structure using the ball and stick model.
So, that's the ball and stick model in a nutshell! Hopefully, this guide has demystified it a bit and you're feeling more confident visualizing molecules. Now go forth and build some awesome structures – and don't be afraid to get a little creative with your ball and stick model creations!