Bacterial Structure: What You Need to Know Now!
Understanding the structure of bacteria is fundamental to various fields, from Microbiology to the development of new antibiotics. A deeper dive into the cell wall as a key component of the structure of bacteria reveals crucial details about bacterial survival and interaction with its environment. Furthermore, Gram staining, a technique routinely performed in labs such as the Centers for Disease Control and Prevention (CDC), helps us classify bacteria based on their structural differences. By grasping these concepts, we unlock the key to better combatting bacterial infections and promoting overall health.
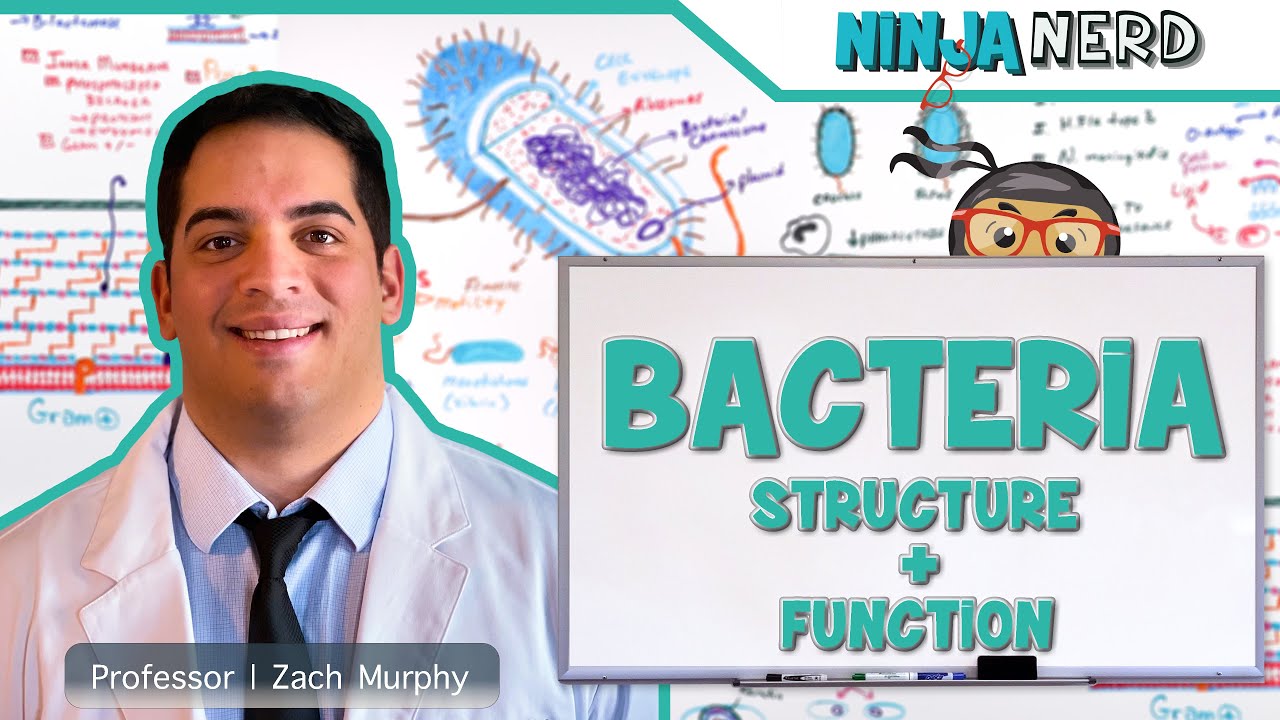
Image taken from the YouTube channel Ninja Nerd , from the video titled Bacteria | Structure and Function .
The world teems with life, a vibrant tapestry woven from organisms both grand and infinitesimal. Among the smallest, yet arguably most influential, are bacteria.
These microscopic entities, often perceived solely through the lens of disease, are in reality vital players in a multitude of ecological processes. They are the unsung heroes of nutrient cycling, the silent partners in our digestion, and the workhorses of numerous biotechnological applications.
Understanding the intricate architecture of these single-celled organisms is paramount. From combating antibiotic resistance to harnessing their potential for groundbreaking innovations, a deep appreciation of bacterial structure is indispensable.
However, our successes in manipulating and controlling bacteria face growing headwinds. The rise of antibiotic resistance presents a formidable challenge, threatening to undo decades of medical progress.
This escalating crisis underscores the urgent need for a renewed focus on understanding the fundamental biology of bacteria, particularly their structural components. By unraveling the secrets of their anatomy, we can pave the way for novel strategies to combat resistant strains and develop more effective therapies.
Why Bacterial Structure Matters
The importance of understanding bacterial structure extends far beyond the realm of medicine. Its significance is deeply intertwined with:
-
Combating Antibiotic Resistance: Deciphering the mechanisms of resistance requires a thorough understanding of the bacterial structures that antibiotics target.
-
Preventing Infectious Diseases: Knowledge of bacterial structure is crucial for developing vaccines and therapies that disrupt bacterial function and prevent infection.
-
Advancing Biotechnology: Bacteria are increasingly being used in a wide range of biotechnological applications, from producing biofuels to synthesizing pharmaceuticals. Optimizing these processes requires a precise understanding of bacterial cell structure.
Bacteria: The Quintessential Prokaryotes
Bacteria belong to a class of organisms known as prokaryotes. Unlike the more complex eukaryotic cells that make up plants, animals, and fungi, prokaryotic cells lack a membrane-bound nucleus and other specialized organelles.
This fundamental difference in cellular organization has profound implications for bacterial structure and function. The absence of a nucleus means that bacterial DNA resides in the cytoplasm, in a region called the nucleoid.
Furthermore, the lack of membrane-bound organelles means that many cellular processes, such as energy production, occur in the cytoplasm or on the cell membrane.
Thesis Statement
This article embarks on a comprehensive exploration of the structural components of bacteria.
By examining each structure in detail, we aim to illuminate its specific function and highlight its overall significance in bacterial physiology, survival, and interactions with the environment.
The Bacterial Fortress: Essential Cellular Components
Having established the paramount importance of bacterial structure, let's embark on a journey into the heart of the bacterial cell. We will explore the fundamental components that underpin its existence.
These essential structures, shared by most bacteria, work in concert to ensure survival, growth, and reproduction. From the rigid cell wall that provides structural integrity to the dynamic cell membrane that regulates the flow of nutrients, each component plays a critical role.
Let's delve into the intricate details of these key players: the cell wall, cell membrane, cytoplasm, ribosomes, DNA, and plasmids. Understanding their individual functions and their complex interdependencies will provide invaluable insights into the inner workings of these microscopic powerhouses.
The Cell Wall: Guardian and Identifier
The bacterial cell wall is a remarkably strong and resilient structure that encases the cell membrane. It acts as the first line of defense against external threats. This essential barrier not only maintains the cell's shape but also prevents it from bursting due to osmotic pressure.
Furthermore, the unique composition of the cell wall is a key characteristic used in bacterial identification, making it a crucial target for diagnostic and therapeutic interventions.
Peptidoglycan: The Foundation of the Fortress
The primary structural component of the bacterial cell wall is peptidoglycan, a complex polymer composed of sugars and amino acids. This mesh-like structure forms a protective layer around the cell, providing rigidity and strength.
The synthesis of peptidoglycan is a complex process involving numerous enzymes. It is a vital target for many antibiotics, such as penicillin, which disrupt its formation and ultimately lead to cell death.
Peptidoglycan’s crucial function is maintaining cell shape. Without it, bacteria would lose their characteristic morphology and become vulnerable to osmotic lysis. This protective role is especially important in hypotonic environments, where water tends to flow into the cell.
Gram-Positive vs. Gram-Negative Bacteria: A Tale of Two Walls
One of the most fundamental distinctions in bacteriology is the classification of bacteria into Gram-positive and Gram-negative based on differences in their cell wall structure. This difference is revealed through the Gram stain procedure, a cornerstone of bacterial identification.
Gram-positive bacteria possess a thick layer of peptidoglycan as the outermost component of their cell wall, while Gram-negative bacteria have a much thinner layer of peptidoglycan sandwiched between an inner cell membrane and an outer membrane. This seemingly small difference has profound implications for antibiotic susceptibility and immune response.
Teichoic and Lipoteichoic Acids in Gram-Positive Bacteria
Embedded within the thick peptidoglycan layer of Gram-positive bacteria are teichoic acids and lipoteichoic acids. These unique molecules play a crucial role in maintaining cell wall stability and regulating cell growth.
Lipoteichoic acids, anchored to the cell membrane, can also elicit an immune response in the host. Their presence contributes to the overall virulence of certain Gram-positive bacteria.
Lipopolysaccharide (LPS) in Gram-Negative Bacteria
The outer membrane of Gram-negative bacteria contains lipopolysaccharide (LPS), a potent endotoxin that triggers a strong immune response in mammals. LPS is composed of three distinct parts: O-antigen, core oligosaccharide, and lipid A.
Lipid A, the toxic component of LPS, is responsible for the fever, inflammation, and septic shock associated with Gram-negative bacterial infections. The O-antigen, on the other hand, is a highly variable region that contributes to the serotyping of bacteria.
The presence of LPS adds another layer of complexity to the Gram-negative cell wall. It makes these bacteria inherently more resistant to certain antibiotics and disinfectants compared to Gram-positive bacteria.
The Cell Membrane: Gatekeeper and Powerhouse
Beneath the cell wall lies the cell membrane, a dynamic and selectively permeable barrier that encloses the cytoplasm. This vital structure serves as the gatekeeper of the cell, controlling the movement of substances in and out.
Beyond its role in transport, the cell membrane is also a powerhouse, playing a critical role in energy production and other essential cellular processes.
The Phospholipid Bilayer and Embedded Proteins
The cell membrane is primarily composed of a phospholipid bilayer, a double layer of lipid molecules with hydrophilic (water-attracting) heads and hydrophobic (water-repelling) tails. This arrangement creates a barrier that is impermeable to most polar molecules.
Embedded within the phospholipid bilayer are a variety of proteins that perform diverse functions. These proteins act as transporters, receptors, enzymes, and structural components. They are crucial for the cell's ability to interact with its environment.
Selective Permeability, Nutrient Uptake, and Waste Excretion
The cell membrane's selective permeability allows it to control which substances enter and exit the cell. This precise regulation is essential for maintaining homeostasis and supporting cellular processes.
Specific transport proteins facilitate the uptake of nutrients, such as sugars and amino acids, while others actively pump out waste products and toxins. This carefully orchestrated exchange ensures that the cell receives the resources it needs and eliminates harmful substances.
Cellular Respiration and ATP Synthesis
In many bacteria, the cell membrane is the site of cellular respiration, the process by which energy is extracted from nutrients. Embedded proteins act as components of the electron transport chain, which generates a proton gradient across the membrane.
This proton gradient is then used by ATP synthase, an enzyme that synthesizes ATP (adenosine triphosphate), the cell's primary energy currency. The cell membrane, therefore, plays a crucial role in powering the cell's activities.
The Cytoplasm: The Hub of Cellular Activity
The cytoplasm is the gel-like substance that fills the interior of the bacterial cell, encompassing all the structures within the cell membrane. It serves as the hub of cellular activity, providing a conducive environment for metabolic reactions, protein synthesis, and other essential processes.
The Cytoplasmic Environment and its Composition
The cytoplasm is primarily composed of water, ions, small molecules, and macromolecules, including proteins, nucleic acids, and lipids. It has a slightly alkaline pH and a high concentration of dissolved solutes.
The cytoplasmic environment is highly organized, with various molecules and structures arranged in specific locations. This spatial organization facilitates efficient biochemical reactions and ensures the proper functioning of the cell.
Hosting Metabolic Reactions and Protein Synthesis
The cytoplasm is the site of numerous metabolic reactions, including glycolysis, the Krebs cycle, and the pentose phosphate pathway. These reactions break down nutrients and generate energy for the cell.
Furthermore, the cytoplasm is where protein synthesis occurs. Ribosomes, the protein synthesis machinery, are located within the cytoplasm, where they translate messenger RNA (mRNA) into proteins.
Ribosomes: The Protein Synthesis Machinery
Ribosomes are essential molecular machines responsible for protein synthesis in all living cells, including bacteria. They translate the genetic code encoded in messenger RNA (mRNA) into functional proteins, which carry out a vast array of cellular functions.
Bacterial ribosomes differ slightly in structure from eukaryotic ribosomes, making them a selective target for certain antibiotics.
Structure and Composition of Bacterial Ribosomes
Bacterial ribosomes are composed of two subunits: a small 30S subunit and a large 50S subunit. Each subunit contains ribosomal RNA (rRNA) molecules and ribosomal proteins.
The 30S subunit is responsible for binding to mRNA and decoding the genetic code, while the 50S subunit catalyzes the formation of peptide bonds between amino acids.
Function in Translating mRNA into Proteins
The process of protein synthesis begins when the 30S subunit binds to mRNA. Transfer RNA (tRNA) molecules, each carrying a specific amino acid, then recognize and bind to the mRNA codons.
The 50S subunit catalyzes the formation of a peptide bond between the amino acids, linking them together to form a polypeptide chain. As the ribosome moves along the mRNA, the polypeptide chain grows longer until a stop codon is reached, signaling the end of translation.
Significance as a Target for Antibiotics
The bacterial ribosome is a prime target for many antibiotics because of its crucial role in protein synthesis and its structural differences from eukaryotic ribosomes.
Antibiotics such as tetracycline and erythromycin bind to specific sites on the bacterial ribosome, interfering with its ability to translate mRNA and synthesize proteins. This ultimately leads to the inhibition of bacterial growth and replication.
DNA (Bacterial Chromosome/Nucleoid): The Genetic Command Center
The bacterial chromosome, also known as the nucleoid, is the cell's genetic command center, housing the DNA that encodes all the information necessary for bacterial survival and reproduction. Unlike eukaryotic cells, bacteria do not have a nucleus. Instead, their chromosome resides in a specific region of the cytoplasm called the nucleoid.
Bacterial Chromosome Structure
The bacterial chromosome is typically a single, circular DNA molecule. This circular chromosome is highly compacted and folded to fit within the confines of the bacterial cell.
Proteins known as nucleoid-associated proteins (NAPs) help to organize and maintain the structure of the bacterial chromosome.
Location within the Nucleoid Region
The nucleoid is not enclosed by a membrane like the nucleus of eukaryotic cells. Instead, it is a distinct region within the cytoplasm that contains the bacterial chromosome and associated proteins.
The nucleoid is a dynamic structure that changes shape and size depending on the cell's growth stage and environmental conditions.
Function in Encoding Genetic Information
The bacterial chromosome contains all the genetic information necessary for cell survival, growth, and reproduction. This information is encoded in the sequence of nucleotide bases (adenine, guanine, cytosine, and thymine) that make up the DNA molecule.
Genes, which are specific segments of DNA, encode the instructions for synthesizing proteins and other essential molecules. The bacterial chromosome, therefore, serves as the blueprint for all cellular processes.
Plasmids: Extra Pieces of the Puzzle
Plasmids are extra-chromosomal DNA molecules found in many bacteria. These small, circular DNA molecules are separate from the bacterial chromosome and can replicate independently.
Plasmids often carry genes that provide bacteria with advantageous traits, such as antibiotic resistance, virulence factors, and the ability to metabolize unusual compounds.
Definition and Types of Plasmids
Plasmids are self-replicating, extra-chromosomal DNA molecules that are typically circular. They range in size from a few thousand base pairs to several hundred thousand base pairs.
There are many different types of plasmids, each carrying a unique set of genes. Resistance plasmids carry genes that confer resistance to antibiotics or other antimicrobial agents. Virulence plasmids carry genes that enhance the bacterium's ability to cause disease.
Role in Horizontal Gene Transfer and Bacterial Adaptation
Plasmids play a critical role in horizontal gene transfer, the process by which bacteria exchange genetic material with each other. This allows bacteria to rapidly acquire new traits, such as antibiotic resistance, without relying on vertical gene transfer (inheritance from parent to offspring).
Plasmids can be transferred between bacteria through various mechanisms, including conjugation, transduction, and transformation. This horizontal gene transfer enables bacteria to adapt quickly to changing environmental conditions.
Importance in Antibiotic Resistance, Virulence, and Other Specialized Functions
The genes carried on plasmids can have a profound impact on bacterial phenotypes. Antibiotic resistance plasmids are a major concern in healthcare, as they contribute to the spread of antibiotic-resistant bacteria.
Virulence plasmids can encode toxins, adhesins, and other factors that enhance a bacterium's ability to infect and cause disease. Plasmids can also carry genes that enable bacteria to metabolize unusual compounds, such as pollutants, making them useful for bioremediation.
Beyond the Basics: Specialized Structures for Survival
Having explored the fundamental components that constitute the bacterial cell, it becomes clear that bacteria are not merely simple bags of enzymes. Their survival hinges on their ability to adapt and thrive in diverse and often hostile environments. Beyond the essential structures, many bacteria possess specialized tools that enhance their survival, virulence, and adaptability.
These fascinating adaptations, like the capsule, flagella, pili, and endospores, provide a competitive edge, enabling bacteria to colonize new niches and resist environmental stressors. Let's delve into these specialized structures, uncovering the secrets behind their remarkable functions and their impact on bacterial life.
The Capsule: The Protective Cloak
The capsule is a layer, often slimy or gummy, that lies outside the cell wall of certain bacteria. Typically composed of polysaccharides, this structure acts as a protective cloak, shielding the bacterium from the host's immune system and environmental hazards.
Capsule Composition and Diversity
The composition of the capsule can vary significantly between different bacterial species. While most capsules are made of polysaccharides, some are composed of polypeptides or even hyaluronic acid. This diversity in composition contributes to the unique properties and functions of the capsule in different bacteria.
Evading the Immune System: A Cloak of Invisibility
One of the primary functions of the capsule is to protect the bacterium from phagocytosis by immune cells. The capsule's slippery surface makes it difficult for phagocytes to engulf and destroy the bacterium, effectively acting as a "cloak of invisibility." This evasion mechanism significantly enhances the bacterium's ability to establish an infection.
Protection Against Desiccation and Environmental Stress
The capsule also plays a vital role in protecting bacteria from desiccation, or drying out. The capsule's hydrophilic nature helps retain moisture around the cell, enabling it to survive in dry or arid environments. Furthermore, the capsule can protect against other environmental stressors, such as extreme temperatures and harmful chemicals.
Virulence and Biofilm Formation: Double-Edged Sword
The capsule's protective properties contribute significantly to the virulence, or disease-causing ability, of many bacteria. By evading the immune system and resisting environmental stressors, encapsulated bacteria are more likely to establish an infection and cause disease.
In addition to its role in virulence, the capsule is also crucial for biofilm formation. Biofilms are communities of bacteria that adhere to surfaces and are encased in a self-produced matrix. The capsule promotes bacterial adhesion and contributes to the structural integrity of the biofilm, making it more resistant to antibiotics and disinfectants.
Flagella: The Engines of Motility
For many bacteria, survival depends on the ability to move and explore their environment. Flagella are whip-like appendages that enable bacteria to swim and migrate towards favorable conditions or away from harmful substances. These remarkable structures are the engines of bacterial motility.
The Intricate Structure of Flagella
The bacterial flagellum is a complex structure comprised of three main components: the filament, the hook, and the basal body.
The filament is the long, helical part that extends from the cell surface and propels the bacterium through its environment. It is composed of flagellin protein subunits.
The hook acts as a flexible joint, connecting the filament to the basal body.
The basal body is embedded in the cell membrane and cell wall. It functions as a rotary motor that drives the rotation of the filament.
The Mechanism of Flagellar Movement
The bacterial flagellum rotates like a propeller, driven by the basal body motor. This motor is powered by the flow of protons (H+) or sodium ions (Na+) across the cell membrane. The direction of flagellar rotation determines the bacterium's movement.
When the flagella rotate counterclockwise, they bundle together and propel the bacterium forward in a smooth, straight line, referred to as a "run."
When the flagella rotate clockwise, they separate and cause the bacterium to tumble randomly, changing its direction. This is called a "tumble."
Motility, Chemotaxis, and Colonization
Flagellar motility is essential for various aspects of bacterial life. It allows bacteria to:
- Seek out nutrients and favorable growth conditions.
- Escape from harmful substances or environmental stressors.
- Colonize new environments and establish infections.
Chemotaxis, the movement of bacteria in response to chemical signals, is crucial for their survival. Bacteria can detect gradients of chemicals in their environment and move towards attractants (e.g., nutrients) or away from repellents (e.g., toxins).
Flagellar motility plays a critical role in the colonization of surfaces and the formation of biofilms. By swimming and attaching to surfaces, bacteria can establish communities and create protective environments for growth and survival.
Pili (Fimbriae): The Attachment Specialists
Pili, also known as fimbriae, are short, hair-like appendages found on the surface of many bacteria. Unlike flagella, pili are not involved in motility but rather serve as attachment specialists, enabling bacteria to adhere to host cells, surfaces, and other bacteria.
Structure and Composition of Pili
Pili are primarily composed of protein subunits called pilins. These pilin subunits assemble to form long, thin filaments that extend from the cell surface. There are different types of pili, each with unique structural and functional properties.
Attachment to Host Cells and Surfaces
The primary function of pili is to mediate the attachment of bacteria to host cells and other surfaces. Pili recognize and bind to specific receptors on host cells, allowing the bacteria to adhere and colonize tissues. This attachment is often the first step in establishing an infection.
Biofilm Formation and Colonization
Pili play a crucial role in the formation of biofilms. By adhering to surfaces and to each other, bacteria can form dense communities encased in a protective matrix. Biofilms are highly resistant to antibiotics and disinfectants, making them a significant challenge in healthcare settings.
Endospores: Dormant Survival Experts
When faced with harsh conditions, such as nutrient deprivation, dehydration, extreme temperatures, or exposure to toxic chemicals, certain bacteria can undergo a remarkable transformation: they form endospores.
Formation and Structure of Endospores
Endospores are dormant, highly resistant structures that enable bacteria to survive for extended periods under unfavorable conditions. The process of endospore formation, called sporulation, involves the replication of the bacterial chromosome. The formation is followed by encapsulation of the chromosome and a small amount of cytoplasm within a tough, protective coat.
The resulting endospore is highly dehydrated and metabolically inactive, making it resistant to heat, radiation, desiccation, and chemical disinfectants.
Survival in Harsh Conditions
Endospores are among the most resistant forms of life known. They can survive for years, decades, or even centuries in a dormant state, waiting for conditions to become favorable again. When conditions improve, the endospore can germinate, giving rise to a new, metabolically active bacterial cell.
Bacillus and Clostridium: Masters of Endospore Formation
Endospore formation is a characteristic feature of certain bacterial genera, most notably Bacillus and Clostridium. These bacteria are commonly found in soil and can cause a variety of diseases, including anthrax (Bacillus anthracis), tetanus (Clostridium tetani), and botulism (Clostridium botulinum).
The ability to form endospores allows these bacteria to persist in the environment for extended periods, increasing their chances of encountering a suitable host and causing disease.
Seeing is Believing: Visualizing Bacterial Structures with Microscopy
Bacteria, with their intricate architectures and sub-cellular components, exist far beyond the reach of the naked eye. To truly understand their form and function, we must turn to the power of microscopy.
Microscopy is the indispensable tool that allows us to peer into the microscopic world, revealing the hidden details of bacterial structure and organization.
Without it, our understanding of bacterial anatomy would remain rudimentary, hindering advancements in medicine, biotechnology, and other crucial fields.
The Power of Magnification
Microscopy allows us to overcome the limitations of human vision, magnifying bacterial cells to a scale where their structural details become discernible.
Different microscopy techniques offer varying degrees of magnification and resolution, each suited to visualizing specific aspects of bacterial anatomy.
From the basic outlines of cell shape to the intricate details of cell wall layers and internal components, microscopy provides the visual evidence necessary to build a comprehensive understanding of bacterial structure.
A Toolkit for Exploring the Microscopic World
Several types of microscopy are routinely employed to study bacterial structures, each with its own strengths and limitations.
Light Microscopy: A Fundamental Approach
Light microscopy, the most basic form, utilizes visible light to illuminate and magnify samples.
It is relatively simple and inexpensive, making it a staple in most microbiology laboratories.
With light microscopy, we can readily observe the shape, size, and arrangement of bacterial cells.
Basic staining techniques can further enhance contrast, allowing us to differentiate between different types of bacteria or highlight specific cellular components.
Electron Microscopy: Unveiling Ultra-Fine Details
Electron microscopy takes magnification to a new level, employing beams of electrons rather than light to create images.
This allows for significantly higher resolution, revealing details that are simply beyond the reach of light microscopy.
There are two main types of electron microscopy: transmission electron microscopy (TEM) and scanning electron microscopy (SEM).
TEM provides detailed images of the internal structures of bacteria, such as cell wall layers, ribosomes, and nucleoids.
Samples must be ultra-thin and specially prepared to allow electrons to pass through.
SEM, on the other hand, provides high-resolution images of the bacterial surface, revealing the texture and arrangement of external structures like flagella and pili.
Fluorescence Microscopy: Illuminating Specific Targets
Fluorescence microscopy employs fluorescent dyes or proteins that selectively bind to specific bacterial components.
When illuminated with light of a particular wavelength, these fluorescent molecules emit light of a different wavelength, creating a colorful and highly specific image.
Fluorescence microscopy is invaluable for visualizing the localization of specific proteins, DNA, or other molecules within bacterial cells.
This technique can be used to study a wide range of cellular processes, from protein synthesis to cell division.
Confocal Microscopy: Building 3D Images
Confocal microscopy is a specialized type of fluorescence microscopy that allows for the creation of three-dimensional images of bacterial structures.
By scanning a focused laser beam across the sample and collecting light from only a single plane of focus, confocal microscopy eliminates out-of-focus blur.
This allows for the construction of detailed 3D reconstructions of biofilms, cells, and other complex bacterial structures.
Examples of Visualized Structures
Each type of microscopy provides unique insights into bacterial structure.
Light microscopy is ideal for observing cell shape (coccus, bacillus, spirillum) and arrangement (chains, clusters).
TEM can reveal the intricate layers of the Gram-positive and Gram-negative cell walls, the internal structure of ribosomes, and the organization of DNA within the nucleoid.
SEM allows us to visualize the distribution and arrangement of flagella and pili on the bacterial surface.
Fluorescence microscopy can highlight the location of specific proteins involved in cell division or antibiotic resistance.
Confocal microscopy allows us to visualize the complex architecture of biofilms, revealing how bacteria are organized and interact within these communities.
In conclusion, microscopy is a cornerstone of bacterial structural biology, providing the means to visualize and understand the intricate details of these microscopic organisms.
By employing a variety of microscopy techniques, researchers can gain a comprehensive understanding of bacterial anatomy, paving the way for new discoveries in medicine, biotechnology, and beyond.
Different microscopy techniques provide us with snapshots of bacterial anatomy, yet they represent only a single moment in a bacterium's life. The bacterial world is far from static. These microorganisms are constantly evolving, adapting, and modifying their structures in response to environmental pressures.
Genes and Form: The Interplay of Bacterial Genetics and Structure
Bacterial structure isn't simply a fixed blueprint; it's a dynamic expression of the organism's genetic code, shaped by evolutionary forces. The genes within a bacterium dictate the construction of its cellular components, but these genes are also subject to mutation and exchange, leading to structural variations that can dramatically impact survival and virulence.
This section explores the intimate relationship between bacterial genetics and structural features, highlighting how genetic mutations and horizontal gene transfer sculpt the bacterial form.
The Genetic Blueprint: Directing Structural Assembly
The bacterial genome contains all the instructions necessary to build and maintain the cell. Genes encode the proteins responsible for synthesizing the cell wall, constructing flagella, assembling ribosomes, and carrying out countless other essential functions.
Therefore, a change in a gene's sequence can have profound consequences for the corresponding structure.
Mutations: Altering the Bacterial Architecture
Genetic mutations, whether spontaneous or induced by external factors, are a primary driver of bacterial evolution. These alterations in the DNA sequence can lead to changes in protein structure and function, ultimately affecting the bacterium's morphology and capabilities.
Mutations Affecting Cell Shape
The shape of a bacterium is largely determined by its cell wall. Mutations in genes involved in peptidoglycan synthesis can disrupt the precise arrangement of this crucial structure, leading to alterations in cell shape.
For example, a mutation might cause a bacterium that is typically rod-shaped (bacillus) to become spherical (coccus). Such changes can influence the bacterium's ability to move, attach to surfaces, or resist environmental stress.
Mutations Affecting Cell Wall Composition
The cell wall's composition is critical for bacterial survival, providing protection and mediating interactions with the environment. Mutations affecting the enzymes involved in peptidoglycan synthesis can alter the cell wall's thickness, cross-linking, or overall integrity.
These changes can affect a bacterium's susceptibility to antibiotics that target the cell wall, such as penicillin and vancomycin.
Mutations Affecting Surface Structures
Surface structures like flagella and pili are essential for bacterial motility, attachment, and biofilm formation. Mutations that disrupt the genes encoding these structures can significantly impair these functions.
A bacterium with a mutated flagellar gene might lose its ability to swim, limiting its ability to colonize new environments. Similarly, mutations in pili genes can prevent bacteria from adhering to host cells, reducing their virulence.
Horizontal Gene Transfer: Acquiring New Structural Traits
In addition to mutations, bacteria can acquire new genetic material through horizontal gene transfer (HGT). This process allows bacteria to exchange genes with other microorganisms, even those of different species, leading to rapid adaptation and the acquisition of novel traits.
Antibiotic Resistance and Cell Wall Modifications
One of the most concerning consequences of HGT is the spread of antibiotic resistance genes. Plasmids, small circular DNA molecules that can be transferred between bacteria, often carry genes that encode resistance mechanisms.
Some resistance genes alter the bacterial cell wall or membrane, making it impermeable to antibiotics or modifying the drug's target site. This can render previously effective antibiotics useless.
Capsule Synthesis and Enhanced Virulence
The capsule, a protective layer surrounding some bacteria, is a key virulence factor that helps bacteria evade the host's immune system. The genes required for capsule synthesis can be transferred horizontally, enabling previously non-encapsulated bacteria to acquire this protective shield.
This acquisition can dramatically enhance the bacterium's ability to cause disease.
In essence, the bacterial form is not static but is perpetually reshaped by its genetic makeup. The continuous dance between genes and structure underscores the remarkable adaptability of bacteria.
Different microscopy techniques provide us with snapshots of bacterial anatomy, yet they represent only a single moment in a bacterium's life. The bacterial world is far from static. These microorganisms are constantly evolving, adapting, and modifying their structures in response to environmental pressures.
Targeting the Enemy: Bacterial Structure as a Key to Antibiotic Development
The intricate architecture of bacteria, meticulously shaped by both genetics and environment, presents a unique vulnerability: its reliance on specific structural components for survival. Understanding these vulnerabilities is paramount to developing effective antibiotics and combating the escalating threat of antibiotic resistance. In essence, a deep knowledge of bacterial structure serves as a blueprint for designing drugs that selectively disrupt essential processes, leading to bacterial cell death or inhibited growth.
Exploiting Structural Weaknesses: A Foundation of Antibiotic Design
The development of effective antibiotics hinges on identifying and exploiting key differences between bacterial cells and host cells. Many successful antibiotics target structures or processes unique to bacteria, minimizing harm to the patient. By understanding the precise molecular mechanisms underlying bacterial structure formation, scientists can design molecules that interfere with these mechanisms, ultimately compromising the bacterium's viability.
This approach requires a thorough understanding of bacterial physiology, biochemistry, and structural biology. It's a multidisciplinary effort, combining insights from diverse fields to develop targeted therapies.
Classic Examples: Antibiotics Targeting Specific Bacterial Structures
Several classes of antibiotics have proven remarkably successful by targeting specific structural components of bacterial cells. Here are some notable examples:
Cell Wall Synthesis Inhibitors: Penicillin and Vancomycin
The bacterial cell wall, a rigid structure essential for maintaining cell shape and withstanding osmotic pressure, is a prime target for antibiotics. Penicillin and other beta-lactam antibiotics inhibit the enzymes responsible for synthesizing peptidoglycan, the primary component of the cell wall.
This weakens the cell wall, leading to cell lysis and death. Vancomycin, another cell wall synthesis inhibitor, binds directly to peptidoglycan precursors, preventing their incorporation into the growing cell wall.
These drugs have been critical in treating bacterial infections for decades.
Ribosome Inhibitors: Tetracycline and Erythromycin
Bacterial ribosomes, the cellular machinery responsible for protein synthesis, differ structurally from eukaryotic ribosomes. This difference allows for the development of antibiotics that selectively target bacterial ribosomes without significantly affecting host cell protein synthesis.
Tetracycline blocks the binding of aminoacyl-tRNA to the ribosome, inhibiting protein synthesis. Erythromycin and other macrolides bind to the ribosome, preventing the translocation step of protein synthesis.
These antibiotics are effective against a wide range of bacterial infections.
DNA Replication Inhibitors: Quinolones
Bacterial DNA replication is another essential process vulnerable to antibiotic attack. Quinolones inhibit DNA gyrase and topoisomerase IV, enzymes responsible for DNA supercoiling and separation during replication.
By interfering with these enzymes, quinolones prevent DNA replication and cell division.
These antibiotics are particularly effective against rapidly dividing bacteria.
The Rising Tide of Resistance: A Call for Innovative Strategies
The success of antibiotics has been tempered by the emergence and spread of antibiotic resistance. Bacteria have evolved various mechanisms to evade the effects of antibiotics, including:
- Enzymatic inactivation of antibiotics: Bacteria produce enzymes that degrade or modify antibiotics, rendering them ineffective.
- Target modification: Bacteria alter the structure of the antibiotic target, preventing the antibiotic from binding.
- Efflux pumps: Bacteria express efflux pumps that actively pump antibiotics out of the cell.
- Reduced permeability: Bacteria decrease the permeability of their cell membranes, preventing antibiotics from entering the cell.
These resistance mechanisms highlight the adaptability of bacteria and the urgent need for innovative strategies to combat antibiotic resistance.
Beyond Traditional Antibiotics: Novel Approaches
Overcoming antibiotic resistance requires a multifaceted approach, including:
- Developing new antibiotics with novel mechanisms of action: This involves identifying new bacterial targets and designing drugs that bypass existing resistance mechanisms.
- Using combination therapy: Combining multiple antibiotics with different mechanisms of action can increase effectiveness and reduce the development of resistance.
- Developing inhibitors of resistance mechanisms: Targeting the enzymes or efflux pumps that confer resistance can restore the effectiveness of existing antibiotics.
- Exploring alternative therapies: Phage therapy, antimicrobial peptides, and immunotherapy offer promising alternatives to traditional antibiotics.
- Structure-Based Drug Design: Utilizing detailed structural information of bacterial proteins to rationally design new drugs.
The battle against antibiotic resistance is an ongoing challenge, but by leveraging our understanding of bacterial structure and function, we can develop new and effective strategies to combat this growing threat. The future of antibiotic development hinges on our ability to exploit the Achilles' heels of bacteria.
Video: Bacterial Structure: What You Need to Know Now!
Bacterial Structure: Frequently Asked Questions
Here are some common questions about the structure of bacteria, helping to clarify key concepts covered in our article.
What are the main structural components of a bacterial cell?
The fundamental structure of bacteria includes a cell wall providing shape and protection, a cell membrane controlling what enters and exits, cytoplasm containing the genetic material (DNA) and ribosomes for protein synthesis. Some bacteria also have additional structures like capsules, flagella, and pili.
How does the bacterial cell wall differ from a plant cell wall?
Bacterial cell walls are primarily composed of peptidoglycan, a unique polymer not found in plant cells. Plant cell walls, on the other hand, are made mainly of cellulose. This difference is crucial because many antibiotics target peptidoglycan synthesis, selectively harming bacteria without affecting our own cells.
What is the role of the bacterial capsule?
The capsule, when present as part of the structure of bacteria, is a slimy outer layer that provides several benefits. It enhances the bacterium's ability to adhere to surfaces, protects it from phagocytosis by immune cells, and helps it resist drying out.
Are all structures of bacteria essential for survival?
Not all structures are essential. While the cell wall, cell membrane, and cytoplasm are crucial for basic survival, structures like flagella and capsules provide advantages in specific environments. The presence or absence of these features influences a bacterium's ability to thrive and cause disease.