Autotrophs: Organisms That Make Their Own Food
Autotrophs, critical to life on Earth, represent the cornerstone of most food chains as organisms that make their own food, converting inorganic substances into energy-rich organic compounds. Photosynthesis, a process primarily utilized by plants in diverse ecosystems, allows these organisms that make their own food to harness light energy from the sun, synthesizing carbohydrates from carbon dioxide and water. The vital role of the organisms that make their own food is exemplified in the research conducted at institutions like the Carnegie Institution for Science, where scientists study the mechanisms of photosynthesis to enhance crop productivity and understand global carbon cycling. Chemosynthesis, an alternative method employed by certain bacteria found near hydrothermal vents, enables these unique organisms that make their own food to derive energy from chemical reactions, independent of sunlight.
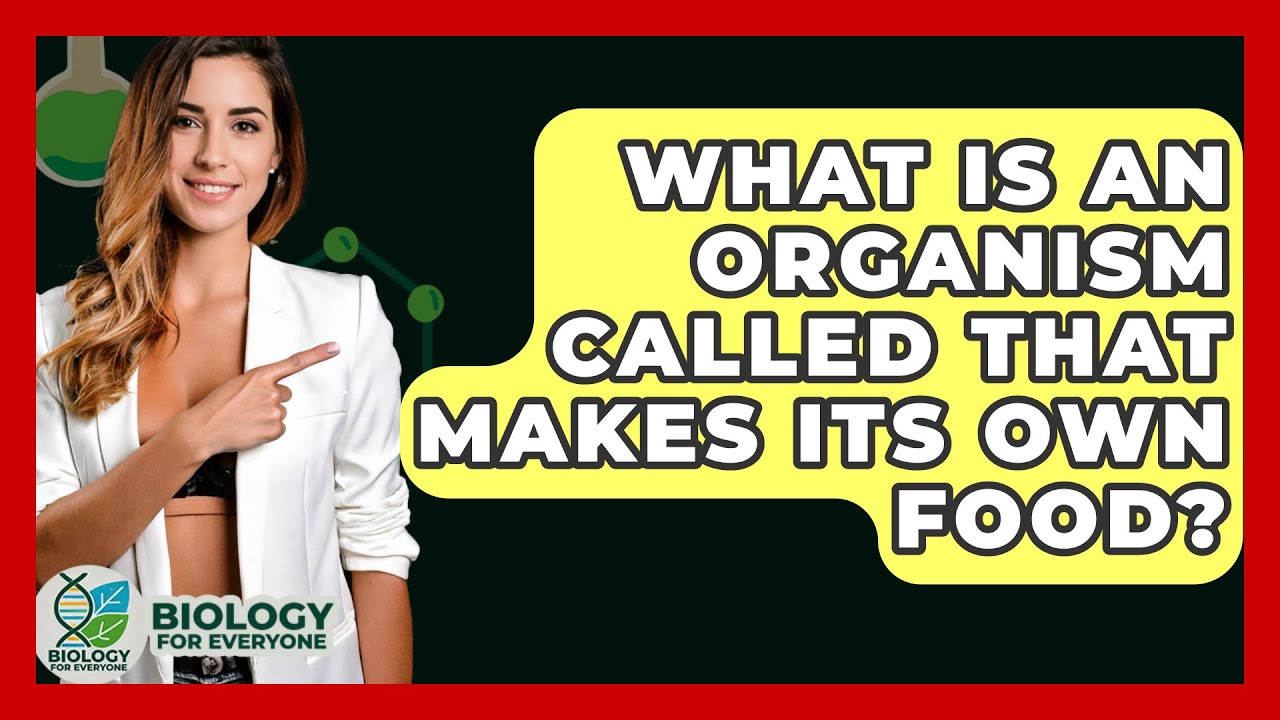
Image taken from the YouTube channel Biology for Everyone , from the video titled What Is An Organism Called That Makes Its Own Food? - Biology For Everyone .
Autotrophs, the self-feeders of the biological world, form the bedrock of virtually all ecosystems. Their unique ability to synthesize organic compounds from inorganic sources distinguishes them as primary producers, fueling life as we know it. Understanding their role and the processes that drive them is paramount to comprehending the intricate web of life on Earth.
Autotrophic Nutrition: Powering Ecosystems
Autotrophic nutrition is the process where organisms create their own food, converting inorganic compounds into energy-rich organic molecules. This remarkable capability allows autotrophs to sustain themselves and, crucially, to provide the energy and nutrients that heterotrophic organisms (those that consume others for sustenance) depend upon. Without autotrophs, the vast majority of food chains and food webs would simply collapse.
Autotrophs are the primary producers in almost every food chain.
They are crucial for building and sustaining ecological stability.
Photosynthesis and Chemosynthesis: Two Paths to Energy
Autotrophs employ two primary strategies for energy production: photosynthesis and chemosynthesis.
Photosynthesis, the more familiar of the two, utilizes sunlight to convert carbon dioxide and water into glucose (a sugar) and oxygen. This process, carried out by plants, algae, and cyanobacteria, is responsible for the vast majority of energy input into terrestrial and aquatic ecosystems.
Chemosynthesis, on the other hand, harnesses the energy from chemical reactions to produce organic compounds. This process is predominantly performed by certain bacteria and archaea, particularly in environments devoid of sunlight, such as deep-sea hydrothermal vents.
The Importance of Understanding Autotrophic Processes
A deep understanding of photosynthesis and chemosynthesis extends far beyond mere academic curiosity.
It has profound implications for various fields, including:
-
Agriculture: Optimizing photosynthetic efficiency in crops can lead to increased yields and food security.
-
Biofuel Production: Harnessing autotrophs (e.g., algae) to produce biofuels offers a sustainable alternative to fossil fuels.
-
Climate Change Mitigation: Understanding and enhancing carbon sequestration by autotrophs can help reduce atmospheric CO2 levels.
The exploration of these processes allows humans to better cope with resource scarcity and environmental problems.
Therefore, continuous research on autotrophs holds the key to a healthier and more sustainable future.
Autotrophs, the self-feeders of the biological world, form the bedrock of virtually all ecosystems. Their unique ability to synthesize organic compounds from inorganic sources distinguishes them as primary producers, fueling life as we know it. Understanding their role and the processes that drive them is paramount to comprehending the intricate web of life on Earth.
Photosynthesis: Harnessing the Power of Sunlight
Building upon the foundational role of autotrophic nutrition, we now delve into one of its most vital mechanisms: photosynthesis. This remarkable process represents the biological world's primary method for capturing the radiant energy of the sun and converting it into a usable form of chemical energy.
Through photosynthesis, organisms like plants, algae, and cyanobacteria act as solar-powered factories, transforming light energy into the very building blocks of life.
The Essence of Photosynthesis
At its core, photosynthesis is the process of using light energy to synthesize sugars (glucose) from carbon dioxide and water. This conversion not only provides the autotroph with the energy it needs to grow and thrive but also generates oxygen as a crucial byproduct.
This oxygen, of course, is essential for the respiration of most life forms on Earth, highlighting the profound impact of photosynthesis on the planet's atmosphere and biodiversity.
The Photosynthetic Trio: Plants, Algae, and Cyanobacteria
While the basic principles of photosynthesis remain consistent, the organisms that employ this process represent a diverse group. Plants, dominating terrestrial landscapes, are perhaps the most familiar photosynthetic organisms.
Their leaves, packed with chloroplasts, are veritable solar panels, efficiently capturing sunlight and converting it into chemical energy.
Algae, inhabiting aquatic environments, are equally important photosynthesizers. Ranging from microscopic single-celled organisms to massive kelp forests, algae contribute significantly to global oxygen production and serve as the foundation of many aquatic food webs.
Cyanobacteria, also known as blue-green algae, are particularly noteworthy from an evolutionary perspective.
These prokaryotic organisms are among the oldest known life forms on Earth and are believed to have played a pivotal role in oxygenating the planet's early atmosphere through their photosynthetic activity.
Their presence and function continues to support life on our planet as we know it.
Products of Photosynthesis: Glucose and Oxygen
The end products of photosynthesis, glucose (a simple sugar) and oxygen, are fundamental to life as we know it. Glucose serves as a readily available source of energy for the autotroph, fueling cellular processes and providing the building blocks for more complex organic molecules.
This means it provides energy for many different organisms.
Oxygen, released into the atmosphere, supports the respiration of aerobic organisms, allowing them to efficiently extract energy from organic compounds. The interplay between photosynthesis and respiration forms a fundamental cycle that sustains life on Earth, with autotrophs capturing solar energy and producing the oxygen that heterotrophs need to survive, and heterotrophs in return, providing carbon dioxide in the atmosphere that autotrophs need.
Autotrophs, the self-feeders of the biological world, form the bedrock of virtually all ecosystems. Their unique ability to synthesize organic compounds from inorganic sources distinguishes them as primary producers, fueling life as we know it. Understanding their role and the processes that drive them is paramount to comprehending the intricate web of life on Earth.
Photosynthesis: Harnessing the Power of Sunlight
Building upon the foundational role of autotrophic nutrition, we now delve into one of its most vital mechanisms: photosynthesis. This remarkable process represents the biological world's primary method for capturing the radiant energy of the sun and converting it into a usable form of chemical energy.
Through photosynthesis, organisms like plants, algae, and cyanobacteria act as solar-powered factories, transforming light energy into the very building blocks of life.
The Essence of Photosynthesis
At its core, photosynthesis is the process of using light energy to synthesize sugars (glucose) from carbon dioxide and water. This conversion not only provides the autotroph with the energy it needs to grow and thrive but also generates oxygen as a crucial byproduct.
This oxygen, of course, is essential for the respiration of most life forms on Earth, highlighting the profound impact of photosynthesis on the planet's atmosphere and biodiversity.
The Photosynthetic Trio: Plants, Algae, and Cyanobacteria
While the basic principles of photosynthesis remain consistent, the organisms that employ this process represent a diverse group. Plants, dominating terrestrial landscapes, are perhaps the most familiar photosynthetic organisms.
Their leaves, packed with chloroplasts, are veritable solar panels, efficiently capturing sunlight and converting it into chemical energy.
Algae, inhabiting aquatic environments, are equally important photosynthesizers. Ranging from microscopic single-celled organisms to massive kelp forests, algae contribute significantly to global oxygen production and serve as the foundation of many aquatic food webs.
Cyanobacteria, also known as blue-green algae, are particularly noteworthy from an evolutionary perspective.
These prokaryotic organisms are among the oldest known life forms on Earth and are believed to have played a pivotal role in oxygenating the planet's early atmosphere through their photosynthetic activity.
Their presence and function continues to support life on our planet as we know it.
Products of Photosynthesis: Glucose and Oxygen
The end products of photosynthesis, glucose (a simple sugar) and oxygen, are fundamental to life as we know it. Glucose serves as a readily available source of energy for the autotroph, fueling cellular processes and providing the building blocks for more complex organic molecules.
This means it provides energy for many different organisms.
Oxygen, released into the atmosphere, supports the respiration of aerobic organisms, allowing them to efficiently extract energy from organic compounds. The interplay between photosynthesis and respiration forms a fundamental cycle that sustains life on Earth, with autotrophs capturing solar energy and producing the oxygen that heterotrophs need to survive, and heterotrophs in return, providing carbon dioxide in the atmosphere that autotrophs need.
Light-Dependent Reactions: Capturing the Sun's Energy
With the groundwork laid regarding the overview of photosynthesis, we now narrow our focus to the initial phase of this process: the light-dependent reactions. This stage is all about capturing the energy of sunlight and converting it into forms that can be used to power the subsequent steps of photosynthesis.
This stage occurs within the thylakoid membranes of the chloroplasts and involves a complex interplay of pigments, proteins, and electron transfer.
The Role of Photosynthetic Pigments
At the heart of light-dependent reactions lies the crucial role of photosynthetic pigments, the molecules responsible for absorbing light energy. Among these, chlorophyll stands out as the primary pigment, giving plants their characteristic green color. Chlorophyll molecules are exceptionally good at absorbing blue and red light, while reflecting green light, which is why we perceive plants as green.
Different types of chlorophyll exist (chlorophyll a, chlorophyll b, etc.), each with slightly different absorption spectra, allowing them to capture a broader range of light wavelengths.
In addition to chlorophyll, carotenoids also play a vital role. These pigments, which include carotenes and xanthophylls, absorb light in the blue-green region of the spectrum. Carotenoids serve two primary functions: they broaden the range of light wavelengths that can be used for photosynthesis, and they also act as antioxidants, protecting chlorophyll from photodamage caused by excessive light intensity.
Think of carotenoids as the supporting cast that enhances the efficiency and resilience of the light-harvesting process.
Photosynthetic pigments are organized into functional units called photosystems, embedded within the thylakoid membranes. There are two main types of photosystems: Photosystem II (PSII) and Photosystem I (PSI).
Each photosystem contains a light-harvesting complex consisting of pigment molecules and proteins that capture light energy and transfer it to a central chlorophyll a molecule in the reaction center.
When light energy reaches the reaction center of PSII, it excites an electron to a higher energy level. This energetic electron is then passed to a series of electron carriers in the electron transport chain (ETC).
PSII, therefore, initiates the process of electron flow that drives the synthesis of ATP.
Electrons leaving PSII are passed down the ETC, eventually arriving at PSI. PSI also absorbs light energy, exciting electrons to an even higher energy level. These energized electrons from PSI are then used to reduce NADP+ to NADPH.
The electron transport chain (ETC) is a series of protein complexes embedded in the thylakoid membrane that plays a critical role in generating ATP and NADPH. As electrons move through the ETC, they release energy, which is used to pump protons (H+) from the stroma into the thylakoid lumen, creating a proton gradient across the thylakoid membrane.
This proton gradient represents a form of potential energy, which is then harnessed by an enzyme called ATP synthase to produce ATP (adenosine triphosphate) through a process called chemiosmosis.
ATP is the primary energy currency of the cell, providing the energy needed for various cellular processes, including the Calvin cycle.
At the end of the electron transport chain associated with Photosystem I, electrons are used to reduce NADP+ (nicotinamide adenine dinucleotide phosphate) to NADPH. NADPH is a reducing agent, meaning it carries high-energy electrons that can be used to reduce (add electrons to) other molecules.
In the context of photosynthesis, NADPH provides the reducing power needed to fix carbon dioxide and synthesize sugars in the Calvin cycle.
In summary, the light-dependent reactions skillfully capture solar energy, convert it into chemical energy in the form of ATP and NADPH, and set the stage for the next phase of photosynthesis, where carbon dioxide is transformed into sugars.
With the energy of sunlight now successfully captured and transformed into ATP and NADPH during the light-dependent reactions, we now transition to the subsequent phase: the Calvin Cycle. This cyclical series of biochemical reactions represents the ultimate destination for that captured energy, where it is used to drive the conversion of carbon dioxide into sugar.
The Calvin cycle, named after Melvin Calvin who mapped the cycle in the 1940s, is where the actual sugar synthesis occurs in photosynthesis.
The Calvin Cycle: Converting Carbon Dioxide into Sugar
The Calvin cycle is a metabolic pathway located in the stroma of the chloroplast, the fluid-filled space surrounding the thylakoids. This is where the "magic" of sugar production truly happens.
It takes the energy harvested in the light-dependent reactions and uses it to "fix" atmospheric carbon dioxide, ultimately building carbohydrate molecules.
Carbon Fixation: The Gateway to Sugar Synthesis
The first major step in the Calvin cycle is carbon fixation. Here, carbon dioxide (CO2) from the atmosphere is combined with a five-carbon molecule called ribulose-1,5-bisphosphate (RuBP).
This reaction is catalyzed by the enzyme ribulose-1,5-bisphosphate carboxylase/oxygenase, more commonly known as RuBisCO. RuBisCO is the most abundant enzyme on Earth, a testament to its critical role in life.
The unstable six-carbon compound that forms immediately breaks down into two molecules of 3-phosphoglycerate (3-PGA). 3-PGA is the first stable organic molecule produced in the Calvin cycle.
Reduction: Building the Sugar
Next comes the reduction phase, where 3-PGA is converted into glyceraldehyde-3-phosphate (G3P), a three-carbon sugar precursor.
This step requires both ATP and NADPH, the energy-rich molecules generated during the light-dependent reactions.
ATP provides the energy for the phosphorylation of 3-PGA, while NADPH provides the reducing power (electrons) needed to reduce the phosphorylated molecule to G3P.
For every six molecules of CO2 that enter the cycle, twelve molecules of G3P are produced. However, only two of these molecules are available for sugar production.
Regeneration: Replenishing the Starting Material
The final phase is regeneration of RuBP. In order for the Calvin cycle to continue functioning, the initial CO2 acceptor, RuBP, must be regenerated.
The remaining ten G3P molecules are used in a complex series of reactions, also requiring ATP, to regenerate six molecules of RuBP.
This ensures that the cycle can continue to fix carbon dioxide and produce more sugar.
This process is like keeping the engine running. Without regeneration, the entire cycle would quickly grind to a halt.
The Stroma: The Stage for the Calvin Cycle
The stroma, as mentioned before, is the fluid-filled space surrounding the thylakoids inside the chloroplasts. This environment provides the ideal conditions for the Calvin cycle enzymes to function.
The stroma contains all the necessary enzymes, substrates, and cofactors required for carbon fixation, reduction, and RuBP regeneration.
It is also where the ATP and NADPH generated by the light-dependent reactions are readily available to power the Calvin cycle.
The Importance of the Electron Transport Chain
While the Calvin cycle itself does not directly involve the electron transport chain, it is entirely dependent on the products of the light-dependent reactions, which are powered by the electron transport chain.
Without the continuous supply of ATP and NADPH from the light-dependent reactions, the Calvin cycle would quickly cease to function.
Therefore, the electron transport chain plays an essential role in indirectly supporting the Calvin cycle and enabling the synthesis of sugars.
In essence, the ETC provides the energy currency for the Calvin cycle to operate.
The Calvin cycle stands as a masterpiece of biochemical engineering, demonstrating how living organisms can capture atmospheric carbon dioxide and transform it into the building blocks of life. This process is central to understanding how autotrophs fuel ecosystems and sustain life on Earth.
With the remarkable processes of light-dependent reactions and the Calvin cycle now elucidated, it's crucial to pinpoint the cellular organelle where this photosynthetic magic truly unfolds: the chloroplast. Understanding the structure and function of chloroplasts is fundamental to grasping the overall mechanism of photosynthesis.
Where Photosynthesis Happens: The Chloroplast
The chloroplast is the defining organelle where photosynthesis takes place in plants and algae. These fascinating structures are more than just green compartments within cells; they are complex, highly organized units specifically designed to capture sunlight and convert it into chemical energy. They are the engines that drive the photosynthetic processes we've discussed.
Chloroplast Structure: A Detailed Look
Chloroplasts possess a distinctive structure that is essential for their function.
Understanding each component sheds light on the overall process of photosynthesis.
Outer and Inner Membranes
Chloroplasts are enclosed by a double membrane, consisting of an outer and inner membrane. These membranes regulate the transport of substances into and out of the chloroplast.
The outer membrane is freely permeable to small molecules, while the inner membrane is more selective, controlling the passage of specific molecules and ions.
The Stroma: The Chloroplast's Cytosol
The stroma is the fluid-filled space within the chloroplast, surrounding the thylakoids.
It contains the enzymes, substrates, and cofactors necessary for the Calvin cycle, where carbon dioxide is fixed and converted into sugars.
Essentially, the stroma is the chloroplast's cytoplasm.
Thylakoids: The Site of Light-Dependent Reactions
Within the stroma lies the thylakoid system: a network of flattened, interconnected sacs called thylakoids.
These thylakoids are the sites of the light-dependent reactions of photosynthesis.
Embedded within the thylakoid membranes are chlorophyll and other pigment molecules that capture light energy.
Grana: Stacks of Thylakoids
Thylakoids are often arranged in stacks called grana (singular: granum).
These stacks increase the surface area available for light absorption, maximizing the efficiency of the light-dependent reactions.
The arrangement ensures optimal light capture and efficient energy transfer.
The Thylakoid Lumen: A Crucial Compartment
The thylakoid membrane encloses an internal space called the thylakoid lumen.
This compartment plays a critical role in ATP synthesis, as it is where a proton gradient is established during the light-dependent reactions.
The proton gradient drives ATP synthase, an enzyme that produces ATP.
The Chloroplast: An Evolutionary Perspective
Interestingly, chloroplasts are believed to have originated from ancient cyanobacteria through a process called endosymbiosis.
This evolutionary history explains why chloroplasts have their own DNA and ribosomes, similar to bacteria.
It's a compelling example of how evolution has shaped the intricate structures within plant cells.
Chloroplasts: Dynamic and Adaptable
Chloroplasts are not static structures.
They can move within the cell to optimize light capture and respond to environmental conditions.
For example, chloroplasts may reposition themselves to avoid excessive light, preventing damage to the photosynthetic machinery.
This adaptability ensures the continued functioning of photosynthesis under varying conditions.
In summary, the chloroplast, with its intricate arrangement of membranes, stroma, thylakoids, and grana, is a highly specialized organelle perfectly suited for carrying out the complex reactions of photosynthesis. Understanding its structure provides invaluable insights into how plants and algae harness the power of sunlight to sustain life on Earth.
Photosynthetic Organisms: A Diverse Group
The world of autotrophs is far from monolithic. The ability to harness light energy for life is spread across a dazzling array of organisms, each uniquely adapted to thrive in specific environments. These photosynthetic powerhouses not only sustain themselves but also form the very foundation of most ecosystems on Earth.
Understanding their diversity and ecological roles is key to appreciating the complexity and interconnectedness of life.
Plants: The Kings of Terrestrial Photosynthesis
Within the Kingdom Plantae lies the vast majority of terrestrial photosynthesizers. From towering redwoods to humble blades of grass, plants have conquered nearly every land-based habitat.
Their success is rooted in their sophisticated photosynthetic machinery and their ability to adapt to diverse environmental conditions.
Plants use chlorophyll to capture sunlight, converting water and carbon dioxide into glucose and oxygen. This process fuels their growth, reproduction, and overall contribution to terrestrial ecosystems.
They are essential primary producers, providing food and habitat for countless other organisms, and also play a crucial role in regulating the Earth's climate.
Algae: Aquatic Photosynthetic Pioneers
Algae, a diverse group spanning various kingdoms including Protista, are the primary photosynthesizers in aquatic environments. They range from microscopic, single-celled organisms to massive kelp forests.
Their photosynthetic capabilities are vital for maintaining aquatic food webs and producing a significant portion of the Earth's oxygen.
Algae exhibit a wide range of adaptations to different light levels, nutrient availability, and water salinities.
Some algae, such as diatoms and dinoflagellates, are single-celled organisms that form the base of many marine food chains, while others, like seaweed, provide important habitats and resources for marine life.
Cyanobacteria: Ancient Photosynthetic Innovators
Cyanobacteria, also known as blue-green algae, are prokaryotic organisms with a fascinating evolutionary history. They were among the first organisms to develop oxygenic photosynthesis, a process that fundamentally altered the Earth's atmosphere.
Their ability to perform photosynthesis allowed them to flourish in a variety of environments, from freshwater lakes to marine ecosystems.
Cyanobacteria play a critical role in nitrogen fixation, converting atmospheric nitrogen into usable forms for other organisms.
Furthermore, they are thought to be the ancestors of chloroplasts, the photosynthetic organelles found in plants and algae, highlighting their profound impact on the evolution of life on Earth.
Phytoplankton: Microscopic Marine Marvels
Phytoplankton are a diverse group of microscopic, photosynthetic organisms that drift in the ocean. Though small, they are incredibly abundant and play a critical role in marine ecosystems.
They form the base of the marine food web, supporting a vast array of organisms, from zooplankton to whales.
Phytoplankton are responsible for a significant portion of the Earth's oxygen production and play a crucial role in regulating the global carbon cycle.
Factors such as nutrient availability, light penetration, and water temperature influence their distribution and productivity.
The balance of phytoplankton communities is crucial to maintaining the health and stability of marine ecosystems. Changes in phytoplankton abundance or composition can have cascading effects throughout the food web.
In conclusion, the diversity of photosynthetic organisms is remarkable. Each group, from plants to phytoplankton, plays a vital role in its respective ecosystem. Understanding their individual contributions and the interconnectedness of these organisms is essential for comprehending the functioning and sustainability of our planet.
Chemosynthesis: Life Without Sunlight
While photosynthesis reigns supreme as the primary engine of life on Earth, a fascinating alternative exists in the form of chemosynthesis. This process, independent of sunlight, allows certain organisms to thrive in environments where light is scarce or entirely absent.
Chemosynthesis represents a remarkable adaptation, showcasing the resilience and ingenuity of life in even the most extreme conditions.
Unveiling Chemosynthesis
Chemosynthesis is the biological process by which certain microorganisms, primarily bacteria and archaea, produce energy by oxidizing inorganic chemical compounds.
Instead of using sunlight as an energy source, these organisms harness the energy stored in chemical bonds of substances like hydrogen sulfide (H₂S), methane (CH₄), ammonia (NH₃), or ferrous iron (Fe²⁺).
This energy is then used to synthesize organic molecules from carbon dioxide (CO₂) or methane, effectively creating their own food source.
Chemoautotrophs: The Key Players
The organisms that perform chemosynthesis are known as chemoautotrophs. These remarkable microbes are the foundation of unique ecosystems, particularly in environments devoid of sunlight, such as deep-sea hydrothermal vents, caves, and subsurface environments.
Chemoautotrophs are broadly classified based on the specific chemical compounds they oxidize:
Sulfur-Oxidizing Bacteria
These bacteria, perhaps the most well-known chemoautotrophs, oxidize sulfur compounds such as hydrogen sulfide (H₂S) to produce energy.
Hydrogen sulfide is often abundant in volcanic regions and deep-sea vents. The energy released from this oxidation is then used to fix carbon dioxide and produce organic matter.
Methane-Oxidizing Bacteria (Methanotrophs)
Methanotrophs consume methane (CH₄) as their primary energy source. They play a crucial role in reducing methane emissions, a potent greenhouse gas, from environments like wetlands and landfills.
These bacteria are active in environments where methane is readily available and contribute significantly to the global carbon cycle.
Nitrifying Bacteria
Nitrifying bacteria are involved in the nitrogen cycle, converting ammonia (NH₃) to nitrite (NO₂⁻) and then to nitrate (NO₃⁻).
This process is essential for soil fertility and plant growth. Two main groups of bacteria are involved: ammonia-oxidizing bacteria and nitrite-oxidizing bacteria.
Iron-Oxidizing Bacteria
These bacteria oxidize ferrous iron (Fe²⁺) to ferric iron (Fe³⁺), obtaining energy from this chemical reaction.
Iron-oxidizing bacteria are commonly found in acidic environments, such as mine drainage sites and iron springs. These bacteria are important for metal cycling in aquatic environments.
Ecological Significance of Chemosynthesis
Chemosynthesis allows life to thrive in environments where photosynthesis is impossible. This is particularly evident in deep-sea hydrothermal vent ecosystems, where entire communities of organisms depend on chemoautotrophic bacteria for their survival.
Tube worms, mussels, and other invertebrates form symbiotic relationships with these bacteria, receiving energy in exchange for providing a suitable habitat and chemical substrates.
Chemosynthesis expands our understanding of the limits of life and highlights the diversity of metabolic strategies organisms employ to survive and thrive on our planet.
Deep-Sea Hydrothermal Vents: Chemosynthesis Hotspots
Deep-sea hydrothermal vents stand as testaments to life's remarkable adaptability. These unique ecosystems, found miles beneath the ocean's surface, thrive in the absence of sunlight, a realm typically considered devoid of life's fundamental energy source. Instead, they are fueled by chemosynthesis, a process that allows life to flourish using the energy derived from chemical reactions.
These vents are not just isolated curiosities; they are vibrant oases teeming with life, challenging our understanding of the boundaries of habitable environments.
The Allure of Hydrothermal Vents
Hydrothermal vents are essentially underwater geysers. They form at tectonic plate boundaries where seawater seeps into the Earth's crust, is superheated by magma, and then re-emerges, laden with dissolved minerals. This creates a chemical-rich environment that supports a diverse array of life.
The extreme conditions of these vents—intense pressure, extreme temperatures, and the absence of sunlight—make them an especially captivating area of study for scientists.
Chemical Energy: Fueling Life in the Dark
The cornerstone of these ecosystems is the chemoautotrophic bacteria and archaea that harness the energy from inorganic chemical compounds. Unlike photosynthetic organisms that rely on sunlight, these microbes oxidize chemicals such as hydrogen sulfide (H₂S), methane (CH₄), and ammonia (NH₃) to produce energy.
This energy is then used to synthesize organic molecules from carbon dioxide or methane, effectively creating their own food source.
Hydrogen Sulfide: A Primary Energy Source
Hydrogen sulfide is one of the most prevalent chemicals used as an energy source in hydrothermal vent ecosystems. Released from the vents, it provides a rich source of electrons for chemosynthetic bacteria.
These bacteria oxidize the hydrogen sulfide, converting it into elemental sulfur or sulfate, and in the process, capturing energy to fuel their metabolic processes.
Other Chemical Energy Sources
While hydrogen sulfide is a major player, other chemicals also contribute to the energy budget of hydrothermal vent ecosystems. Methane, released from the Earth’s interior, is another important energy source that is consumed by methanotrophic bacteria, which play a crucial role in the local carbon cycle.
Ammonia is also utilized by certain chemoautotrophs, further diversifying the range of metabolic strategies that support these unique environments.
Symbiotic Relationships: A Key to Survival
Many of the larger organisms found at hydrothermal vents, such as tube worms, mussels, and clams, have developed symbiotic relationships with chemoautotrophic bacteria.
These invertebrates provide the bacteria with a safe habitat and a constant supply of chemicals from the vent fluids. In return, the bacteria provide the host organism with the organic compounds they synthesize through chemosynthesis. This partnership allows both organisms to thrive in the harsh vent environment.
The discovery and study of deep-sea hydrothermal vents have profoundly impacted our understanding of life on Earth, demonstrating the remarkable resilience of life and the potential for life to exist in other extreme environments throughout the universe.
Primary Production: The Foundation of Every Ecosystem
The flow of energy through ecosystems begins with primary production, a process of paramount importance to all life on Earth. This foundational process dictates the availability of energy for all subsequent trophic levels, shaping the structure and function of entire communities. Without it, ecosystems, as we know them, could not exist.
Primary production is essentially the creation of organic compounds from atmospheric or aquatic carbon dioxide. This creation is driven by autotrophs, the self-feeders of the biological world, which employ either photosynthesis or chemosynthesis to achieve this remarkable feat.
Defining Primary Production
At its core, primary production refers to the rate at which autotrophs convert inorganic carbon into organic matter. This rate can be expressed as the amount of carbon fixed per unit area or volume per unit time.
Primary production is further subdivided into:
- Gross Primary Production (GPP): The total rate of carbon fixation by autotrophs.
- Net Primary Production (NPP): The rate of carbon fixed by autotrophs, minus their respiration losses. NPP represents the energy available to higher trophic levels. It’s the energy passed up the food chain.
NPP is a critical metric because it reflects the actual amount of energy available to support consumers, decomposers, and ultimately, the entire ecosystem.
Photosynthesis and Chemosynthesis: Twin Pillars of Primary Production
Both photosynthesis and chemosynthesis play vital roles in primary production, although they operate in vastly different environments and employ distinct energy sources.
- Photosynthesis, the dominant process, utilizes sunlight to convert carbon dioxide and water into glucose and oxygen. This process fuels the vast majority of terrestrial and aquatic ecosystems. The sunlight creates the energy, and the energy then fuels the reaction.
- Chemosynthesis, on the other hand, harnesses the energy from chemical reactions, such as the oxidation of hydrogen sulfide, methane, or ammonia. This process is particularly important in environments devoid of sunlight, such as deep-sea hydrothermal vents and subsurface environments. Here the oxidation, in turn, drives the energy production.
The Role of Primary Production in Carbon Sequestration
The ability of autotrophs to fix carbon dioxide from the atmosphere or oceans is a cornerstone of carbon sequestration. Through photosynthesis and chemosynthesis, these organisms act as significant carbon sinks, removing CO2 from the environment and incorporating it into their biomass.
This process helps regulate the concentration of greenhouse gases in the atmosphere, mitigating the effects of climate change. In this cycle, not only is the environment becoming more sustainable, but so is the biological chain.
When autotrophs die and decompose, some of this stored carbon is released back into the atmosphere through respiration, while some is buried in sediments, contributing to long-term carbon storage.
Understanding the factors that influence primary production is therefore crucial for predicting and managing the global carbon cycle.
Ecosystem Services and Primary Production
Primary production underpins a wide range of ecosystem services that are essential for human well-being.
- Food production: All agriculture relies on photosynthetic primary production.
- Oxygen production: Photosynthesis releases oxygen as a byproduct, maintaining the atmospheric oxygen levels necessary for animal life.
- Water purification: Plants and algae help filter and purify water.
- Climate regulation: Through carbon sequestration, primary producers help regulate Earth's climate.
- Habitat provision: Primary producers form the base of food webs, supporting diverse communities of organisms.
The health and productivity of ecosystems are directly linked to the rate of primary production. Maintaining and enhancing primary production is, therefore, essential for ensuring the sustainability of these vital ecosystem services.
Environmental Contexts: Where Autotrophs Thrive
The remarkable ability of autotrophs to synthesize their own food allows them to thrive in a diverse range of environments, each presenting unique challenges and opportunities. From the sun-drenched canopies of rainforests to the dark, abyssal depths of hydrothermal vents, autotrophs have evolved fascinating strategies to harness energy and sustain life.
Photosynthetic Paradises: Sunlight-Driven Ecosystems
Tropical Rainforests: Hubs of Photosynthetic Activity
Tropical rainforests represent the pinnacle of photosynthetic productivity on Earth. The combination of consistently high temperatures, abundant rainfall, and intense sunlight creates ideal conditions for rapid plant growth.
The dense vegetation, characterized by towering trees, sprawling vines, and a multitude of epiphytes, captures a significant portion of the incoming solar radiation, fueling a complex and highly productive ecosystem.
The sheer biomass of rainforests makes them critical players in the global carbon cycle, absorbing vast quantities of CO2 from the atmosphere.
Oceanic Zones: The Realm of Phytoplankton
The photic zone of the ocean, the uppermost layer penetrated by sunlight, teems with microscopic photosynthetic organisms known as phytoplankton. These tiny algae and cyanobacteria form the base of the marine food web, supporting a vast array of marine life.
Phytoplankton are responsible for a significant portion of global photosynthesis, contributing an estimated 50% of the oxygen in Earth's atmosphere.
Factors such as nutrient availability, water temperature, and light intensity influence phytoplankton productivity, leading to seasonal blooms and regional variations in primary production.
Salt Marshes: Coastal Autotrophs
Salt marshes, located in coastal intertidal zones, are dynamic ecosystems characterized by salt-tolerant plants known as halophytes. These plants, such as cordgrass (Spartina) and glasswort (Salicornia), have adapted to survive in high-salinity environments, using specialized mechanisms to regulate salt uptake and maintain osmotic balance.
Salt marshes are highly productive ecosystems, providing crucial habitat for a variety of invertebrates, fish, and birds.
They also play a vital role in coastal protection, buffering shorelines from erosion and storm surges.
Deserts: Adapting to Aridity
Deserts, characterized by low rainfall and extreme temperatures, present formidable challenges for photosynthetic organisms.
However, a variety of plants have evolved remarkable adaptations to thrive in these arid conditions. These adaptations include:
- Succulence (water storage).
- Deep root systems.
- Reduced leaf surface area.
- Specialized photosynthetic pathways such as CAM (crassulacean acid metabolism).
CAM plants, such as cacti and succulents, open their stomata at night to absorb CO2, minimizing water loss during the heat of the day.
Arctic Tundra: Resilience in the Cold
The Arctic tundra, a cold and treeless biome, is characterized by permafrost, low temperatures, and a short growing season.
Despite these harsh conditions, a variety of autotrophs, including lichens, mosses, and dwarf shrubs, manage to survive and contribute to primary production.
Lichens, symbiotic associations between fungi and algae or cyanobacteria, are particularly well-adapted to the tundra environment, able to withstand extreme cold and desiccation.
They play an essential role in nutrient cycling and soil stabilization in these fragile ecosystems.
Chemosynthetic Sanctuaries: Life in the Dark
Deep-Sea Hydrothermal Vents: Oases of Chemosynthesis
Deep-sea hydrothermal vents are unique ecosystems found along mid-ocean ridges, where tectonic plates are spreading apart.
These vents release superheated water and chemicals, such as hydrogen sulfide, methane, and ammonia, from the Earth's interior.
Chemoautotrophic bacteria and archaea thrive in these dark and extreme environments, utilizing the energy from these chemicals to synthesize organic matter through chemosynthesis.
These chemosynthetic microbes form the base of the food web, supporting a diverse community of organisms, including tube worms, clams, and crustaceans.
The discovery of hydrothermal vent ecosystems revolutionized our understanding of life on Earth, demonstrating that life can exist independently of sunlight.
Historical Perspectives: Key Discoveries
The story of understanding autotrophs is a testament to human curiosity and the relentless pursuit of knowledge. Our current comprehension of photosynthesis and chemosynthesis is built upon the foundation laid by numerous scientists whose groundbreaking experiments and insightful observations unlocked the secrets of how life sustains itself.
Unraveling the Calvin Cycle: A Nobel-Winning Achievement
Melvin Calvin's work stands as a monumental achievement in the history of photosynthesis research. In the late 1940s and early 1950s, Calvin, along with his team at the University of California, Berkeley, meticulously traced the path of carbon during photosynthesis using radioactive carbon-14 as a tracer.
The "Lollipop" Experiment
Their now-famous "lollipop" experiment involved exposing Chlorella algae to radioactive carbon dioxide for short periods, then quickly halting the process and analyzing the compounds that had incorporated the radioactive carbon. By identifying the sequence in which different compounds became radioactive, Calvin and his colleagues were able to elucidate the cyclic pathway of carbon fixation.
This intricate cycle, now known as the Calvin Cycle, describes how carbon dioxide is converted into glucose within the chloroplasts of plant cells. This discovery, earned Melvin Calvin the Nobel Prize in Chemistry in 1961.
Andrew Benson's Crucial Role
While Melvin Calvin rightfully receives the majority of the recognition for the Calvin Cycle, the contributions of Andrew Benson were equally critical. Benson was instrumental in identifying ribulose-1,5-bisphosphate (RuBP) as the initial carbon dioxide acceptor molecule in the cycle.
His expertise in carbohydrate chemistry and his dedication to experimental rigor were essential to unraveling the complex reactions involved in carbon fixation. Benson's collaboration with Calvin exemplifies the power of teamwork in scientific discovery. Their combined insights laid the groundwork for subsequent research that has further refined our understanding of photosynthetic carbon metabolism.
The Quest for Artificial Photosynthesis
Inspired by the elegance and efficiency of natural photosynthesis, scientists have long sought to replicate this process artificially. The goal of artificial photosynthesis is to develop synthetic systems that can capture sunlight and convert it into chemical energy, such as hydrogen or other fuels.
This pursuit holds immense potential for addressing global energy challenges and mitigating climate change. Artificial photosynthesis aims to create sustainable and carbon-neutral energy sources.
Current Research Efforts
Current research in artificial photosynthesis focuses on several key areas, including:
-
Developing efficient light-harvesting materials: Creating synthetic analogs of chlorophyll and other pigments to capture a broader spectrum of sunlight.
-
Designing catalysts for water splitting: Developing catalysts that can efficiently split water into hydrogen and oxygen using solar energy.
-
Engineering electron transfer pathways: Mimicking the electron transport chains of natural photosynthesis to efficiently transfer electrons and generate energy.
-
Creating integrated systems: Combining light-harvesting materials, catalysts, and electron transfer components into functional artificial photosynthetic devices.
While significant challenges remain, the field of artificial photosynthesis is rapidly advancing. Continued research in this area promises to unlock innovative solutions for a sustainable energy future, inspired by the remarkable processes that sustain life on Earth.
Tools and Techniques: Studying Autotrophic Processes
Unraveling the intricate mechanisms of photosynthesis and chemosynthesis requires a sophisticated arsenal of tools and techniques. Scientists employ a diverse range of methods to probe the inner workings of autotrophs, from analyzing the pigments that capture light to tracing the flow of carbon through metabolic pathways. These techniques provide invaluable insights into how these processes function and how they are influenced by environmental factors.
Spectrophotometry: Unveiling the Secrets of Pigments
Spectrophotometry is a cornerstone technique for analyzing the pigments involved in photosynthesis. This method measures the absorption and transmission of light through a sample.
By examining the spectral properties of pigments like chlorophylls and carotenoids, scientists can determine their concentration and identify their specific types. Each pigment has a unique absorption spectrum, acting like a fingerprint that reveals its identity.
Spectrophotometry is critical for understanding how light energy is captured and utilized in photosynthetic organisms. It also enables researchers to assess the health and productivity of plant and algal communities.
Gas Chromatography-Mass Spectrometry (GC-MS): Identifying Metabolic Compounds
To dissect the complex web of biochemical reactions in autotrophs, scientists rely on Gas Chromatography-Mass Spectrometry (GC-MS). This powerful analytical technique separates and identifies different compounds within a sample.
GC-MS is particularly useful for studying the products and intermediates of photosynthesis and chemosynthesis. It can identify various organic acids, sugars, and other metabolites involved in carbon fixation and energy production.
By tracing the flow of carbon through these compounds, scientists can map out the metabolic pathways and understand how they are regulated. GC-MS is, therefore, an indispensable tool for studying autotrophic metabolism.
Microscopy: Visualizing Cellular Structures
Microscopy provides a window into the cellular world of autotrophs, allowing scientists to visualize the structures involved in photosynthesis and chemosynthesis. Light microscopy offers a broad view of cells and tissues, revealing the distribution of chloroplasts in plant cells or the morphology of chemosynthetic bacteria.
Electron microscopy, with its much higher resolution, allows for detailed examination of subcellular structures. Transmission electron microscopy (TEM) can reveal the intricate architecture of thylakoid membranes within chloroplasts, while scanning electron microscopy (SEM) can provide three-dimensional images of cell surfaces and microbial communities.
Both light and electron microscopy are essential for understanding the spatial organization of autotrophic processes and how they relate to cellular function.
Stable Isotope Analysis: Tracing Carbon Flow
Stable isotope analysis is a powerful method for tracing the flow of carbon through ecosystems. This technique relies on the fact that different isotopes of carbon (such as 12C and 13C) are processed slightly differently by biological organisms.
By measuring the ratios of these isotopes in different organisms and environmental samples, scientists can track the origin and fate of carbon.
This is particularly useful for understanding the relative contributions of photosynthesis and chemosynthesis to primary production in different ecosystems. Stable isotope analysis can reveal the complex food web interactions that support life in diverse environments, from tropical rainforests to deep-sea hydrothermal vents.
Applications and Implications: Real-World Impact
The fundamental processes of photosynthesis and chemosynthesis are not confined to the realm of academic study. Instead, these autotrophic pathways underpin a wide array of practical applications that directly impact our lives and shape our future.
Understanding the intricate mechanisms by which autotrophs convert energy into usable forms unlocks opportunities for enhancing crop yields, developing sustainable energy sources, mitigating climate change, and even sustaining life in the most challenging environments, including space.
Agriculture: Photosynthesis for Food Security
Photosynthesis is the foundation of nearly all food chains on Earth. Optimizing this process in crops is essential for ensuring global food security.
By understanding the factors that limit photosynthetic efficiency, such as light availability, CO2 concentration, and nutrient supply, we can develop strategies to enhance crop productivity.
These strategies include:
- Developing crop varieties with improved photosynthetic capacity.
- Optimizing irrigation and fertilization practices.
- Employing techniques like controlled environment agriculture (CEA).
- Genetic modification (GM) to create crops that photosynthesize more efficiently.
Biofuel Production: Autotrophs as Renewable Energy Factories
Autotrophs, particularly algae and certain bacteria, offer a promising avenue for sustainable biofuel production. These organisms can efficiently convert sunlight or chemical energy into biomass rich in lipids and carbohydrates, which can then be processed into biofuels like biodiesel and bioethanol.
Compared to traditional biofuel sources like corn and soybeans, autotrophic biofuel production has several advantages:
- Higher yields per unit area.
- Reduced competition with food crops.
- The ability to utilize non-arable land and wastewater.
Research in this area focuses on optimizing autotrophic growth conditions, enhancing lipid production, and developing efficient biofuel conversion technologies.
Carbon Sequestration: Autotrophs as Climate Change Allies
Autotrophs play a crucial role in the global carbon cycle by absorbing atmospheric CO2 during photosynthesis and chemosynthesis. Enhancing this natural carbon sequestration capacity is a key strategy for mitigating climate change.
Forests, oceans, and soils act as major carbon sinks, with photosynthetic organisms like trees, phytoplankton, and algae driving carbon uptake.
Strategies to enhance carbon sequestration include:
- Reforestation and afforestation efforts.
- Sustainable land management practices.
- Ocean fertilization to promote phytoplankton growth.
- Developing technologies to capture CO2 directly from the atmosphere and utilize it for algal biofuel production.
Ecosystem Services: Autotrophs as Life Support Systems
Autotrophs provide a wealth of ecosystem services that are essential for maintaining life on Earth.
These include:
- Oxygen production.
- Water purification.
- Soil formation.
- Climate regulation.
By supporting primary production, autotrophs form the base of food webs, providing energy and nutrients for all other organisms. Protecting and restoring autotroph-dominated ecosystems, such as forests, wetlands, and coral reefs, is, therefore, vital for maintaining biodiversity and human well-being.
Climate Change Mitigation: Autotrophs in Global Carbon Cycling
Understanding the role of autotrophs in global carbon cycling is crucial for predicting and mitigating the impacts of climate change.
Photosynthesis and chemosynthesis are key drivers of carbon fluxes between the atmosphere, oceans, and land. Changes in these processes, driven by factors like deforestation, ocean acidification, and nutrient pollution, can have profound consequences for global climate.
Research efforts are focused on:
- Developing models to predict how autotrophic activity will respond to climate change.
- Identifying strategies to enhance carbon sequestration in different ecosystems.
- Reducing emissions of greenhouse gases that disrupt the balance of the carbon cycle.
Space Exploration: Autotrophs as Extraterrestrial Life Support
The ability of autotrophs to convert inorganic matter into food and oxygen makes them invaluable for long-duration space missions and the establishment of extraterrestrial settlements.
Photosynthetic organisms can be integrated into closed-loop life support systems to regenerate air, purify water, and produce food for astronauts.
Research in this area focuses on:
- Developing efficient bioreactors for growing plants and algae in space.
- Identifying autotrophic species that can thrive in the harsh conditions of space.
- Engineering autotrophs to produce essential nutrients and pharmaceuticals.
Interdisciplinary Connections: A Web of Knowledge
The study of autotrophs, organisms capable of synthesizing their own food through photosynthesis or chemosynthesis, is far from a solitary pursuit. Rather, it represents a vibrant nexus where diverse scientific disciplines converge. Understanding these interconnected relationships is vital for a complete and nuanced appreciation of the roles and impacts of autotrophs within our world.
Botany: Unveiling the Plant Kingdom
Botany, the scientific study of plants, forms a cornerstone in understanding photosynthesis. Plants, belonging to the Kingdom Plantae, are the dominant terrestrial photosynthesizers, responsible for a significant portion of global primary production.
Botanists delve into the intricate details of plant physiology, anatomy, and genetics to unravel the mechanisms that govern photosynthesis.
Research in this field focuses on optimizing plant growth, enhancing photosynthetic efficiency, and developing crop varieties that are more resilient to environmental stresses.
Furthermore, botany explores the evolutionary history of photosynthesis, tracing its origins and diversification across the plant kingdom.
Microbiology: Exploring the Microscopic World of Autotrophs
Microbiology plays a crucial role in illuminating the world of autotrophic microorganisms, including cyanobacteria (blue-green algae) and various other bacteria and archaea.
Cyanobacteria, for instance, are ancient photosynthetic organisms that played a pivotal role in oxygenating Earth's atmosphere.
Microbiologists investigate the metabolic pathways, genetic makeup, and ecological interactions of these microorganisms.
This research is essential for understanding the role of microbial autotrophs in nutrient cycling, biogeochemical processes, and the development of novel biotechnologies.
Ecology: Understanding Ecosystem Dynamics
Ecology, the study of the interactions between organisms and their environment, provides a critical framework for understanding the role of autotrophs in ecosystem dynamics. Autotrophs, as primary producers, form the base of food webs, converting energy from sunlight or chemical compounds into biomass that supports all other organisms.
Ecologists study the distribution, abundance, and productivity of autotrophs in different ecosystems, examining how these factors are influenced by environmental conditions and species interactions.
Understanding these dynamics is crucial for managing and conserving ecosystems, as well as for predicting the impacts of environmental change on primary production.
Moreover, ecological studies help to elucidate the complex relationships between autotrophs and other organisms, such as herbivores, decomposers, and symbiotic partners.
Biochemistry: Decoding the Chemical Reactions of Life
Biochemistry provides the essential tools and knowledge for unraveling the intricate chemical reactions that underlie photosynthesis and chemosynthesis.
Biochemists investigate the structure and function of enzymes, pigments, and other molecules involved in these processes.
By elucidating the metabolic pathways and regulatory mechanisms, biochemistry sheds light on how autotrophs capture and convert energy, synthesize organic compounds, and respond to environmental cues.
This knowledge is invaluable for optimizing autotrophic processes for applications such as biofuel production and carbon sequestration.
Molecular Biology: Unraveling the Molecular Mechanisms
Molecular biology delves into the molecular mechanisms that govern photosynthesis and chemosynthesis, exploring the genes, proteins, and regulatory elements that control these processes.
Molecular biologists use techniques such as gene sequencing, gene editing, and protein engineering to manipulate autotrophic organisms and enhance their performance.
This research is leading to the development of crops with improved photosynthetic efficiency, algae with enhanced lipid production for biofuel, and microorganisms capable of removing pollutants from the environment.
Furthermore, molecular biology is providing insights into the evolutionary origins of photosynthesis and chemosynthesis, revealing how these processes have adapted and diversified over time.
In conclusion, the study of autotrophs is inherently interdisciplinary, requiring the combined expertise of botanists, microbiologists, ecologists, biochemists, and molecular biologists. By integrating these diverse perspectives, we can gain a deeper understanding of the fundamental processes that sustain life on Earth and develop innovative solutions to address pressing challenges such as food security, climate change, and sustainable energy.
Contrasting Processes: Different Strategies for Life
The remarkable ability of autotrophs to synthesize their own food through photosynthesis or chemosynthesis stands in stark contrast to the nutritional strategies employed by other life forms. Understanding these contrasting processes illuminates the diverse ways organisms obtain energy and nutrients, highlighting the fundamental differences between autotrophic and heterotrophic lifestyles.
Autotrophs vs. Heterotrophs: A Tale of Two Trophies
Autotrophs, derived from the Greek words "autos" (self) and "trophe" (nourishment), are the self-feeders of the biological world. They occupy the crucial role of primary producers, converting inorganic compounds into organic matter, the building blocks of life. Through photosynthesis, plants, algae, and cyanobacteria harness solar energy to convert carbon dioxide and water into glucose and oxygen. Chemosynthetic bacteria, on the other hand, derive energy from chemical reactions, oxidizing inorganic compounds such as hydrogen sulfide or ammonia to produce organic molecules.
In contrast, heterotrophs, meaning "other-nourishment," cannot synthesize their own food and must obtain organic compounds by consuming other organisms or organic matter. This group encompasses animals, fungi, and most bacteria. Heterotrophs rely directly or indirectly on autotrophs for their energy and nutrients, forming complex food webs that underpin ecosystem stability. The fundamental distinction lies in the source of carbon: autotrophs obtain carbon from inorganic sources (CO2), while heterotrophs obtain carbon from organic sources (other organisms).
Photosynthesis and Cellular Respiration: A Reciprocal Relationship
Photosynthesis and cellular respiration represent two opposing yet complementary processes that drive the flow of energy and matter in ecosystems. Photosynthesis, as previously discussed, captures light energy and converts it into chemical energy in the form of glucose, releasing oxygen as a byproduct.
Cellular respiration, on the other hand, is the process by which organisms break down glucose and other organic molecules to release energy in the form of ATP (adenosine triphosphate), the energy currency of cells. This process consumes oxygen and releases carbon dioxide and water as waste products.
The relationship between photosynthesis and respiration is cyclical. The products of photosynthesis (glucose and oxygen) are the reactants of cellular respiration, and the products of cellular respiration (carbon dioxide and water) are the reactants of photosynthesis. This reciprocal exchange of gases and energy creates a delicate balance that sustains life on Earth.
Deep Dive Into Heterotrophic Nutrition
Heterotrophic nutrition encompasses a wide array of feeding strategies, each adapted to exploit different food sources. These strategies can be broadly categorized into:
- Herbivores: Consume plants (e.g., cows, deer, caterpillars).
- Carnivores: Consume other animals (e.g., lions, sharks, spiders).
- Omnivores: Consume both plants and animals (e.g., humans, bears, crows).
- Decomposers: Consume dead organic matter (e.g., fungi, bacteria, earthworms).
Each feeding strategy is associated with specific adaptations, such as specialized digestive systems, hunting techniques, or scavenging behaviors.
Contrasting Respiration with Photosynthesis
While photosynthesis uses light energy to create glucose from carbon dioxide and water, respiration breaks down glucose to release energy for cellular activities. These processes also differ in their location, with photosynthesis occurring in chloroplasts and respiration occurring in the cytoplasm and mitochondria of cells. The products of photosynthesis, oxygen and glucose, are reactants of respiration, while the waste products of respiration, water and carbon dioxide, are the reactants of photosynthesis.
In summary, autotrophs and heterotrophs employ fundamentally different strategies for obtaining energy and nutrients. Autotrophs create their own food using photosynthesis or chemosynthesis, while heterotrophs obtain food by consuming other organisms. Photosynthesis and cellular respiration are reciprocal processes that drive the flow of energy and matter in ecosystems. Understanding these contrasting processes is essential for comprehending the intricate web of life and the interconnectedness of all living organisms.
Video: Autotrophs: Organisms That Make Their Own Food
FAQs About Autotrophs
What is an autotroph?
An autotroph is an organism that produces its own food using light, water, carbon dioxide, or other chemicals. These organisms that make their own food are also called producers, forming the base of most food chains.
How do autotrophs make their own food?
Autotrophs utilize processes like photosynthesis and chemosynthesis to create energy-rich molecules. Photosynthesis uses sunlight, while chemosynthesis uses chemicals from the environment to power the creation of food by these organisms that make their own food.
What are some examples of autotrophs?
Plants are a common example, using photosynthesis. Algae and certain bacteria are also autotrophs. These organisms that make their own food include any living thing capable of creating its own energy source.
Why are autotrophs important?
Autotrophs are vital because they convert inorganic compounds into organic compounds, which are essential for all other life forms. They are the foundation of ecosystems, providing the energy needed for organisms that make their own food and all other organisms higher up the food chain.
So, next time you're enjoying a salad or just breathing the fresh air, take a moment to appreciate the amazing world of autotrophs – those incredible organisms that make their own food. They're the foundation of almost every ecosystem and truly make life as we know it possible. Pretty cool, right?