ATP vs ADP Structure: Energy Currency Explained
ATP and ADP, the ubiquitous energy currencies within cellular biology, dictate the flow of energy in every living organism from bustling mitochondria in human cells to the simplest bacteria. The phosphate groups, pivotal components of ATP and ADP, influence the molecule's energy storage capacity and functionality, dictating whether a molecule is an energy source or a discharged unit, which is central to understanding the difference in atp vs adp structure. Adenosine, a nucleoside, serves as the foundational building block for both molecules, yet the variance in the number of phosphate groups attached radically alters its role in cellular processes and the overall energy budget managed by systems such as Krebs Cycle.
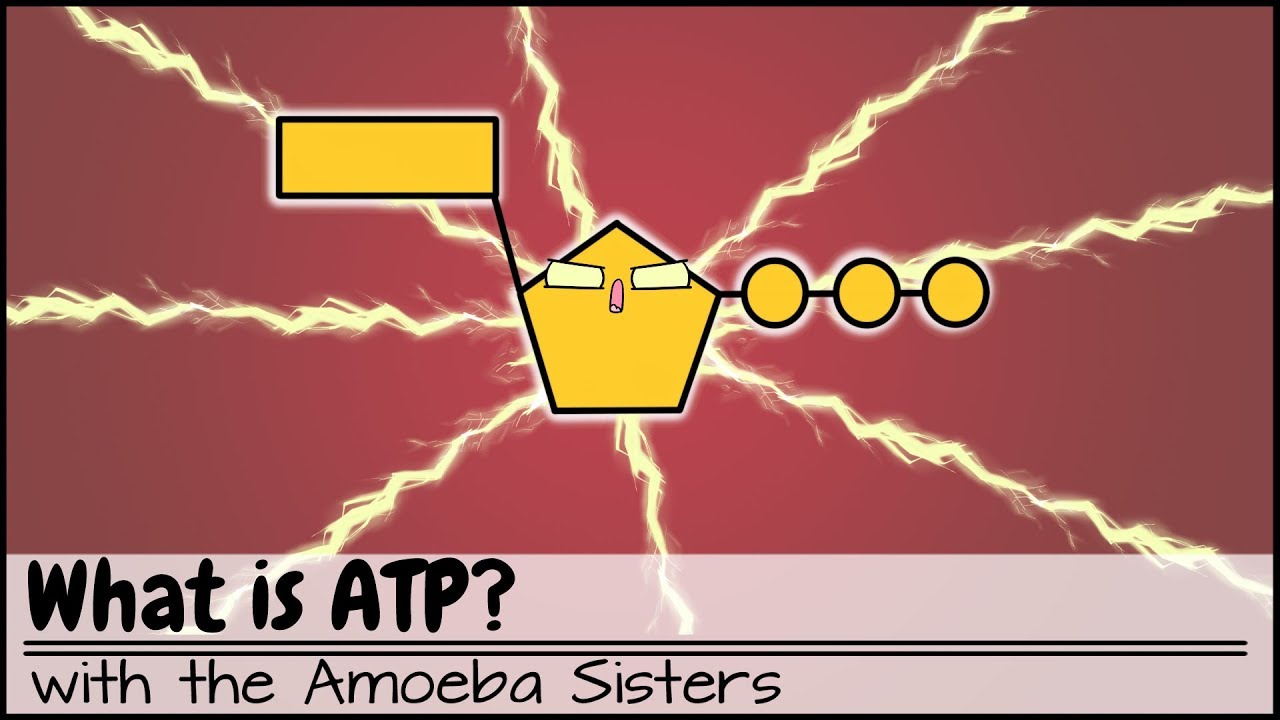
Image taken from the YouTube channel Amoeba Sisters , from the video titled What is ATP? .
ATP: The Universal Energy Currency of Life
Life, in all its magnificent forms, dances to the tune of energy. And the conductor of this intricate energetic symphony is Adenosine Triphosphate, or ATP. This remarkable molecule isn't just another component of living cells; it's the very fuel that powers them.
Without ATP, the cellular processes that sustain life would grind to a halt.
What Exactly is ATP?
ATP is a complex organic chemical. At its core, ATP is an adenosine molecule (composed of adenine and ribose) bound to three phosphate groups. It's a nucleotide, similar in structure to the building blocks of DNA and RNA, but with a far more dynamic and immediate role.
Think of ATP as a tiny, rechargeable battery, readily available to provide energy on demand.
The Vital Role of ATP
ATP's fundamental role lies in its ability to store and release energy for cellular work. From muscle contraction to nerve impulse transmission, from protein synthesis to active transport across cell membranes, ATP is the driving force.
Virtually every energy-requiring process within a cell relies directly on ATP.
ATP is involved in:
- Mechanical Work: Powering muscle contraction and cellular movement.
- Transport Work: Moving molecules across cell membranes against their concentration gradients.
- Chemical Work: Supplying the energy needed for endergonic reactions, such as synthesizing complex molecules.
ATP, ADP, and AMP: A Tale of Energy Transfer
To truly understand ATP, it's helpful to consider its close relatives: ADP (Adenosine Diphosphate) and AMP (Adenosine Monophosphate). These molecules are structurally similar to ATP but contain fewer phosphate groups.
The key difference lies in their energy levels.
ATP holds the most energy, followed by ADP, and then AMP. The conversion between these forms is central to energy transfer within cells.
When ATP is hydrolyzed (broken down by water), it loses one phosphate group, becoming ADP and releasing energy. This energy fuels cellular processes. Conversely, ADP can be phosphorylated (a phosphate group is added) to regenerate ATP, replenishing the energy supply. AMP can also be converted to ADP, and then to ATP.
This continuous cycle of ATP breakdown and regeneration ensures a constant supply of energy. By understanding ATP’s structure, function, and interconversion with ADP and AMP, we can begin to unravel the elegant mechanisms that sustain life at the cellular level.
Molecular Architecture: Deconstructing the ATP Molecule
Having established ATP as the cell's primary energy currency, let's delve into its molecular architecture. Understanding the structural components of ATP is crucial to appreciating how it performs its vital role. ATP isn't just a random assortment of atoms; it's a carefully constructed molecule designed for efficient energy storage and release.
Think of it as a finely tuned engine, each part contributing to its overall performance.
The Three Pillars of ATP: Ribose, Adenine, and the Triphosphate Tail
ATP, short for Adenosine Triphosphate, comprises three essential components: a ribose sugar, an adenine base, and a triphosphate group. These three components interlink to allow ATP to function as the energy currency of the cell.
Ribose: The Sugar Backbone
The ribose sugar forms the structural backbone of ATP. This five-carbon sugar, also found in RNA, provides the foundation upon which the other components are attached. It's the anchor point for both the adenine base and the crucial triphosphate tail.
Adenine: The Identifying Marker
The adenine base is a nitrogenous compound that is also a component of DNA. Its presence defines the molecule as an adenosine derivative, distinguishing it from other nucleotide-based energy carriers. While adenine doesn't directly participate in energy storage, it’s essential for ATP's recognition by enzymes and cellular machinery.
The Triphosphate Tail: The Energy Powerhouse
The triphosphate tail is where the magic truly happens. This chain of three phosphate groups is linked together by phosphoanhydride bonds, which are the key to ATP's energy storage capabilities. These bonds are high-energy bonds, meaning they require a significant amount of energy to form, and they release a substantial amount of energy when broken.
Phosphoanhydride Bonds: The Secret to ATP's Energy Storage
The phosphoanhydride bonds are the heart of ATP's energy-storing capacity. These bonds, connecting the phosphate groups in the triphosphate tail, are inherently unstable. The negative charges of the phosphate groups repel each other, creating a state of high potential energy.
Breaking these bonds, through a process called hydrolysis, releases that stored energy, providing the power that drives cellular processes.
This instability, while seemingly a weakness, is actually ATP's greatest strength. It allows for a rapid and controlled release of energy precisely when and where it's needed.
Phosphate (Pi): The Versatile Player in Cellular Regulation
When ATP is hydrolyzed, one phosphate group is cleaved off, releasing energy and forming ADP (Adenosine Diphosphate). The released phosphate group, often denoted as Pi (inorganic phosphate), isn't just a byproduct; it plays a crucial role in regulating various cellular processes.
Pi can bind to other molecules, a process called phosphorylation, altering their activity and driving conformational changes. This mechanism is essential for signal transduction, enzyme regulation, and a multitude of other cellular functions.
In essence, phosphate acts as a molecular switch, turning processes on or off in response to cellular needs. The careful control of phosphorylation and dephosphorylation (the removal of phosphate) is fundamental to cellular homeostasis.
Understanding the molecular architecture of ATP, from its ribose backbone to its energy-rich triphosphate tail, provides a solid foundation for appreciating its dynamic role in cellular energy transfer. Now, let's explore the dynamic processes of ATP hydrolysis and phosphorylation.
The ATP Cycle: Hydrolysis, Phosphorylation, and Dephosphorylation
ATP doesn't just passively store energy; it actively participates in a continuous cycle of energy transfer. This dynamic cycle revolves around three key processes: hydrolysis, phosphorylation, and dephosphorylation. Understanding these interconnected reactions is paramount to grasping how ATP fuels life at the molecular level.
ATP Hydrolysis: Unleashing the Energy Within
ATP hydrolysis is the process by which ATP is broken down, releasing energy. This reaction involves the breaking of one of the phosphoanhydride bonds in the triphosphate tail. Critically, this process requires water; hence the term "hydrolysis," meaning "splitting with water."
The chemical equation for ATP hydrolysis is straightforward: ATP + H2O → ADP + Pi + Energy.
The crucial role of water cannot be overstated. A water molecule attacks the terminal phosphate group, breaking the bond and releasing a phosphate ion (Pi) and adenosine diphosphate (ADP). This seemingly simple reaction unleashes a surge of energy that the cell can then harness to perform work.
This energy release isn't just heat; it's carefully channeled to power a myriad of cellular activities, from muscle contraction to protein synthesis. Without ATP hydrolysis, the cell would grind to a halt.
Phosphorylation: Activating Cellular Processes
While hydrolysis is about releasing energy, phosphorylation is about using that energy to activate other molecules. Phosphorylation is the process of adding a phosphate group (derived from ATP hydrolysis) to another molecule, typically a protein.
This addition can dramatically alter the target molecule's shape, and therefore its function. Think of it like flipping a switch; the phosphate group acts as a molecular on/off switch, triggering a cascade of downstream events.
Many enzymes, signaling proteins, and structural components within the cell are regulated via phosphorylation. Kinases are the enzymes responsible for carrying out phosphorylation reactions. They are key regulators of cellular activity, adding phosphate groups to specific target proteins.
Through phosphorylation, ATP indirectly fuels countless biological processes, ensuring that the cell can respond dynamically to its environment.
Dephosphorylation: Reversing the Activation
The activation of proteins and other molecules through phosphorylation is not a permanent state. Dephosphorylation, the removal of a phosphate group from a molecule, is just as critical for cellular regulation.
Phosphatases are the enzymes responsible for catalyzing this dephosphorylation process. These enzymes counteract the effects of kinases, removing phosphate groups and returning the target molecule to its original, inactive state.
Dephosphorylation acts as a molecular "off" switch. By removing the phosphate group, the molecule reverts to its original conformation, thus terminating its activity or signaling. This process allows for fine-tuned control over cellular pathways, preventing them from running indefinitely.
The interplay between phosphorylation and dephosphorylation is a fundamental regulatory mechanism, allowing cells to respond rapidly and precisely to changing conditions. This dynamic equilibrium ensures that cellular processes are neither perpetually active nor inactive, but rather finely tuned to meet the cell's needs.
In essence, the coordinated actions of kinases and phosphatases govern the flow of information and energy within the cell, creating a dynamic and responsive system.
Cellular Power Plants: Pathways to ATP Production
Following the dynamic interplay of ATP hydrolysis and phosphorylation, a critical question arises: where does all this ATP come from? The answer lies within the cellular power plants – the metabolic pathways meticulously designed to synthesize ATP, ensuring a constant supply of this essential energy currency. Two primary pathways reign supreme: cellular respiration and photosynthesis.
Cellular Respiration: Harvesting Energy from Glucose
Cellular respiration is the maestro of ATP production in most organisms, from single-celled bacteria to complex multicellular animals. It's a carefully orchestrated series of biochemical reactions that extract energy from glucose (or other organic molecules) and convert it into the usable form of ATP.
This process unfolds in several key stages, each contributing to the overall ATP yield.
Glycolysis: The Initial Glucose Breakdown
Glycolysis, meaning "sugar splitting," is the first act in cellular respiration. Occurring in the cytoplasm, this pathway breaks down one molecule of glucose into two molecules of pyruvate.
While glycolysis itself generates a small amount of ATP directly, its primary role is to prepare pyruvate for the subsequent stages. This process also produces NADH, a crucial electron carrier.
The Krebs Cycle (Citric Acid Cycle): Oxidizing Fuel Molecules
Pyruvate, generated from glycolysis, enters the mitochondria where it's converted into Acetyl-CoA.
Acetyl-CoA then enters the Krebs Cycle (also known as the Citric Acid Cycle), a cyclic series of reactions that further oxidizes the molecule, releasing carbon dioxide and generating more electron carriers (NADH and FADH2).
The Krebs Cycle itself produces a limited amount of ATP directly, but its main contribution lies in the abundant generation of these energy-rich electron carriers.
The Electron Transport Chain: A Cascade of Electron Transfer
The NADH and FADH2 molecules, brimming with high-energy electrons, deliver their cargo to the electron transport chain (ETC) located in the inner mitochondrial membrane.
Here, electrons are passed down a series of protein complexes, releasing energy at each step. This energy is then used to pump protons (H+) across the membrane, creating an electrochemical gradient.
Oxidative Phosphorylation: Harnessing the Proton Gradient
Oxidative phosphorylation is the grand finale of cellular respiration, where the energy stored in the proton gradient is finally harnessed to synthesize ATP.
The enzyme ATP synthase acts as a channel, allowing protons to flow back down their concentration gradient, effectively converting the potential energy of the gradient into the kinetic energy of a spinning rotor. This mechanical energy then drives the phosphorylation of ADP to form ATP. This process, known as chemiosmosis, is remarkably efficient and generates the vast majority of ATP during cellular respiration.
Photosynthesis: Capturing Sunlight's Energy
While cellular respiration is the primary ATP-generating pathway for most organisms, photosynthesis reigns supreme in the plant kingdom (and some bacteria and protists). This remarkable process captures light energy from the sun and converts it into chemical energy in the form of glucose and, ultimately, ATP.
Plants employ specialized pigments, such as chlorophyll, to absorb sunlight. This captured energy is then used to split water molecules, releasing electrons and generating ATP and NADPH (another important electron carrier).
The ATP produced during the "light-dependent reactions" of photosynthesis is then used to fuel the "light-independent reactions" (Calvin cycle), where carbon dioxide is fixed and converted into glucose. This glucose can then be used as fuel for the plant's own cellular respiration, providing a sustainable energy source.
In essence, photosynthesis is the ultimate source of energy for most life on Earth, converting solar energy into the chemical energy that sustains ecosystems. The constant production of ATP is fundamental to life on earth.
ATP in Action: Fueling Cellular Processes
ATP isn't just a molecule sitting idly by; it's a dynamic player, the prime mover in countless cellular events. It’s the cellular equivalent of ready-to-use cash, instantly accessible to drive reactions that would otherwise be thermodynamically unfavorable. Let's dive into how ATP directly fuels life's processes.
ATP Powers Endergonic Reactions: Overcoming Energy Barriers
Cells constantly perform endergonic reactions – processes that require an input of energy to proceed. Think of building complex molecules, transporting substances against their concentration gradients, or powering muscle contraction. These reactions simply cannot happen spontaneously.
This is where ATP steps in as the ultimate energy donor.
The key lies in energetic coupling.
Specifically, the exergonic (energy-releasing) hydrolysis of ATP is coupled to the endergonic reaction.
Think of it as ATP "paying" for the reaction to occur. The energy released from breaking the phosphoanhydride bond is directly used to drive the endergonic reaction forward.
For example, consider the synthesis of glutamine from glutamic acid and ammonia. This reaction is endergonic and requires energy. By coupling it with ATP hydrolysis, the overall reaction becomes thermodynamically favorable, allowing glutamine to be synthesized.
This precise energy transfer is critical for countless biosynthetic pathways.
ATP Hydrolysis: The Energy Currency Exchange
While ATP excels at powering endergonic reactions, it's vital to understand that ATP hydrolysis is the process that yields the usable energy. Hydrolysis refers to the chemical breakdown of a compound due to reaction with water.
When ATP is hydrolyzed, it breaks down into ADP (adenosine diphosphate) and inorganic phosphate (Pi), releasing energy in the process. This energy release isn't just a random event; it's a carefully controlled transfer of chemical potential energy into kinetic or other forms of energy that the cell can harness.
The change in free energy (ΔG) for ATP hydrolysis is negative, indicating that it is an exergonic reaction, meaning it releases energy. It is this released energy that fuels a multitude of cellular processes.
Enzymes: The Catalysts of ATP Reactions
ATP hydrolysis doesn't just happen on its own. Like any biochemical reaction, it typically requires a catalyst to speed up the reaction rate and ensure it occurs efficiently within the cellular environment. This is where enzymes come into play.
Enzymes are biological catalysts, typically proteins, that dramatically accelerate the rate of biochemical reactions without being consumed in the process. In the case of ATP, specific enzymes bind to ATP and the target molecule, facilitating the transfer of the phosphate group and the release of energy.
These enzymes, often called kinases, are highly specific and play crucial roles in signal transduction, metabolic regulation, and many other cellular processes. They lower the activation energy required for the reaction to proceed, allowing it to occur much faster than it would otherwise.
Furthermore, enzymes are not just passive facilitators; they also provide a controlled microenvironment that optimizes the reaction conditions. This ensures that the energy released from ATP hydrolysis is directed precisely to the intended target, maximizing efficiency and minimizing unwanted side reactions.
Location Matters: The Organelles of ATP Synthesis
ATP synthesis isn't a free-for-all happening randomly throughout the cell. This vital process is meticulously organized and compartmentalized within specific organelles. These organelles are specifically adapted to the task, providing the ideal environment and machinery for efficient ATP production.
Let's explore these crucial locations, the mitochondria and chloroplasts, the unsung heroes of cellular energy generation.
Mitochondria: The Powerhouses of Eukaryotic Cells
Mitochondria, often dubbed the "powerhouses of the cell," are membrane-bound organelles found in the vast majority of eukaryotic cells.
These remarkable structures are the primary sites of cellular respiration, the process by which glucose and other fuel molecules are broken down to generate ATP. Their intricate architecture is perfectly suited for maximizing ATP production.
The Mitochondrial Structure: Optimized for Energy Production
Mitochondria possess a distinctive double-membrane structure. The outer membrane is relatively smooth, while the inner membrane is highly folded, forming structures called cristae. This infolding dramatically increases the surface area available for the electron transport chain and ATP synthase, the key players in oxidative phosphorylation.
The space between the two membranes is called the intermembrane space, and the space enclosed by the inner membrane is the mitochondrial matrix.
Each compartment plays a vital role in the stepwise production of ATP.
Cellular Respiration: A Multi-Step ATP Symphony
Within the mitochondria, the Krebs cycle (or citric acid cycle) takes place in the matrix, generating electron carriers (NADH and FADH2) that fuel the electron transport chain.
This chain is embedded in the inner mitochondrial membrane and harnesses the energy from these electron carriers to pump protons (H+) across the membrane, creating an electrochemical gradient.
The potential energy stored in this gradient is then used by ATP synthase to drive the synthesis of ATP from ADP and inorganic phosphate, in a process called chemiosmosis.
The strategic location of these processes within the mitochondria is essential for efficient energy conversion.
Chloroplasts: Harnessing Sunlight for ATP Production
In the plant kingdom, chloroplasts reign supreme as the sites of photosynthesis. These organelles, found in plant cells and algae, are responsible for capturing light energy from the sun and converting it into chemical energy in the form of ATP and other energy-rich molecules like NADPH.
This ATP, generated during the light-dependent reactions of photosynthesis, is then used to power the light-independent reactions (Calvin cycle), where carbon dioxide is converted into glucose.
The Chloroplast Structure: Solar Energy Converters
Like mitochondria, chloroplasts also possess a double-membrane structure. Within the inner membrane lies a complex network of interconnected sacs called thylakoids. These thylakoids are often stacked into structures called grana.
The thylakoid membranes contain chlorophyll and other pigments that absorb light energy, while the space surrounding the thylakoids is called the stroma.
The intricate arrangement provides a vast surface area for capturing sunlight and carrying out the photosynthetic reactions.
Photosynthesis: From Sunlight to Chemical Energy
During the light-dependent reactions, light energy is absorbed by chlorophyll and used to split water molecules, releasing electrons, protons, and oxygen.
The electrons are passed along an electron transport chain within the thylakoid membrane, generating a proton gradient across the membrane.
Similar to mitochondria, this proton gradient is then used by ATP synthase to produce ATP via chemiosmosis.
The ATP, along with NADPH (another energy-carrying molecule), is then utilized in the stroma during the Calvin cycle to fix carbon dioxide and produce sugars.
The strategic location of these processes within the chloroplasts is critical for harnessing solar energy and converting it into usable chemical energy for the plant.
In summary, the carefully organized and specialized environments within mitochondria and chloroplasts demonstrate the elegance and efficiency of cellular energy production. These organelles are far more than just locations; they are integral components of the ATP synthesis machinery, essential for sustaining life as we know it.
Key Players in ATP Production: ATP Synthase and Chemiosmosis
While we've discussed the organelles where ATP is synthesized, the actual machinery and mechanism powering this synthesis deserve closer scrutiny.
Two critical components stand out: ATP synthase and chemiosmosis.
These are not merely players; they are the star performers in the ATP production drama.
ATP Synthase: The Molecular Turbine
ATP synthase is a remarkable enzyme, arguably one of the most important proteins in all of biology.
Imagine a tiny molecular turbine, spinning and churning out ATP molecules with incredible efficiency.
That's essentially what ATP synthase does.
This complex protein structure is found embedded in the inner mitochondrial membrane of eukaryotes and the thylakoid membrane of chloroplasts in plants.
Its primary function? To catalyze the synthesis of ATP from ADP and inorganic phosphate.
Structure and Function: A Rotary Engine
ATP synthase is composed of two main subunits: F0 and F1.
The F0 subunit is embedded within the membrane and acts as a channel for protons (H+) to flow across.
The F1 subunit protrudes into the mitochondrial matrix or the chloroplast stroma and contains the catalytic sites for ATP synthesis.
As protons flow through the F0 channel, they cause it to rotate, like water turning a turbine.
This rotation drives conformational changes in the F1 subunit, which then binds ADP and inorganic phosphate together to form ATP.
It's a stunning example of how mechanical energy can be directly converted into chemical energy at the molecular level!
The efficiency of ATP synthase is truly astounding.
Each complete rotation of the F0 subunit can result in the synthesis of several ATP molecules.
This makes it one of the most efficient molecular machines known to science.
Chemiosmosis: The Proton Gradient Power Source
ATP synthase doesn't work in isolation.
It relies on a crucial prerequisite: a proton gradient across the membrane.
This is where chemiosmosis comes in.
Chemiosmosis is the process by which the movement of ions (in this case, protons) across a selectively permeable membrane down their electrochemical gradient is coupled to the synthesis of ATP.
Think of it as building up a dam and then using the rushing water to turn a turbine.
Building the Gradient: Pumping Protons
In mitochondria, the electron transport chain uses the energy from electron carriers (NADH and FADH2) to pump protons from the mitochondrial matrix into the intermembrane space.
This creates a high concentration of protons in the intermembrane space and a low concentration in the matrix, establishing an electrochemical gradient.
In chloroplasts, a similar process occurs in the thylakoid membrane during the light-dependent reactions of photosynthesis.
Light energy drives the pumping of protons from the stroma into the thylakoid lumen, creating a proton gradient.
Harnessing the Gradient: ATP Production
The proton gradient represents a form of potential energy.
The protons "want" to flow back across the membrane to equalize the concentration.
However, the membrane is impermeable to protons except through the ATP synthase channel.
As protons flow down their concentration gradient through ATP synthase, the energy released is used to drive ATP synthesis.
This coupling of proton flow to ATP synthesis is the essence of chemiosmosis.
In conclusion, ATP synthase and chemiosmosis are inseparable partners in ATP production.
ATP synthase acts as the molecular turbine, while chemiosmosis provides the proton gradient "fuel" that drives the turbine.
Together, they represent a sophisticated and efficient system for converting energy into the universal currency of life: ATP.
Video: ATP vs ADP Structure: Energy Currency Explained
FAQs: ATP vs ADP Structure - Energy Currency Explained
What is the key structural difference between ATP and ADP?
ATP (adenosine triphosphate) and ADP (adenosine diphosphate) differ in the number of phosphate groups. ATP has three phosphate groups attached to adenosine, while ADP has only two. This difference in phosphate groups is central to understanding the atp vs adp structure and their roles.
How does the atp vs adp structure relate to energy storage?
ATP stores energy in the bonds connecting its phosphate groups. When a phosphate group is broken off from ATP, forming ADP, energy is released for cellular processes. The atp vs adp structure dictates how much potential energy is stored within each molecule.
Can ADP be converted back to ATP?
Yes, ADP can be converted back to ATP through the process of phosphorylation, which adds a phosphate group back onto the ADP molecule. This requires energy input, often from the breakdown of food. This cyclical atp vs adp structure conversion is essential for energy flow in cells.
Why is understanding atp vs adp structure important?
Understanding the atp vs adp structure is fundamental to understanding how cells store and use energy. The structure determines the energy capacity and how it's released to power vital functions such as muscle contraction, protein synthesis, and nerve impulse transmission.
So, next time you're feeling that burst of energy after a snack, remember those little ATP vs ADP structure changes happening on a molecular level! It's pretty amazing how such a simple difference in a phosphate group can fuel everything we do.